Difference between revisions of "Coastal and marine sediments"
Dronkers J (talk | contribs) (Created page with " {{Definition|title=Sediment |definition= Natural unconsolidated granular material with density greater than water.}} ==Origin of coastal and marine sediments== Continent...") |
Dronkers J (talk | contribs) |
||
(27 intermediate revisions by the same user not shown) | |||
Line 1: | Line 1: | ||
− | {{Definition|title= | + | |
− | |definition= Natural unconsolidated granular material with density greater than water.}} | + | {{Definition|title=Marine sediment |
+ | |definition= Natural unconsolidated granular material with sediment density greater than water.}} | ||
Line 7: | Line 8: | ||
==Origin of coastal and marine sediments== | ==Origin of coastal and marine sediments== | ||
− | Continental shelves are formed of sediment deposits | + | Continental shelves are formed for the major part from deposits of sediment discharged by rivers. These deposits can reach a thickness of more than 1 km. The sediments have different origins: |
===Clastic sediments <ref name=H>Huggett, R.J. 2007. Fundamentals of geomorphology. Routledge, Taylor & Francis</ref>=== | ===Clastic sediments <ref name=H>Huggett, R.J. 2007. Fundamentals of geomorphology. Routledge, Taylor & Francis</ref>=== | ||
− | Clastic sediments (clast = fragment) are terrigenous materials eroded from land. | + | Clastic sediments (clast = fragment) are ultimate weathering products derived from rock. There are many types of rock, which differ by their origin, crystalline structure and mineral composition: |
+ | * Igneous rock = solidified magma. [https://en.wikipedia.org/wiki/Felsic Felsic rock] (e.g., [https://en.wikipedia.org/wiki/Granite ''granite''], [https://en.wikipedia.org/wiki/Rhyolite ''rhyolite'']) is rich in [https://en.wikipedia.org/wiki/Quartz quartz], [https://en.wikipedia.org/wiki/Orthoclase orthoclase feldspar], [https://en.wikipedia.org/wiki/Amphibole amphibole], [https://en.wikipedia.org/wiki/Muscovite muscovite], [https://en.wikipedia.org/wiki/Biotite biotite]; [https://en.wikipedia.org/wiki/Mafic Mafic rock] (e.g., [https://en.wikipedia.org/wiki/Gabbro ''gabbro''], [https://en.wikipedia.org/wiki/Basalt ''basalt'']) is rich in [https://en.wikipedia.org/wiki/Olivine olivine], [https://en.wikipedia.org/wiki/Pyroxene pyroxene], [https://en.wikipedia.org/wiki/Anorthite anorthite feldspar]. | ||
+ | * Sedimentary rock = lithified deposits of mineral or organic particles (e.g., [https://en.wikipedia.org/wiki/Breccia ''breccia''], [https://en.wikipedia.org/wiki/Gritstone ''gritstone''], [https://en.wikipedia.org/wiki/Conglomerate_(geology) ''conglomerate''], [https://en.wikipedia.org/wiki/Diamictite ''diamict''], [https://en.wikipedia.org/wiki/Sandstone ''sandstone''], [https://en.wikipedia.org/wiki/Greywacke ''greywacke''], [https://en.wikipedia.org/wiki/Arkose ''arkose''], [https://en.wikipedia.org/wiki/Flagstone ''flagstone''], [https://en.wikipedia.org/wiki/Siltstone ''siltstone''], [https://en.wikipedia.org/wiki/Mudstone ''mudstone''], [https://en.wikipedia.org/wiki/Marl ''marl'']) | ||
+ | * Metamorphic rock = sedimentary rock transformed under great heat and pressure beneath the earth crust (e.g., [https://en.wikipedia.org/wiki/Gneiss ''gneiss''], [https://en.wikipedia.org/wiki/Slate ''slate''], [https://en.wikipedia.org/wiki/Marble ''marble''], [https://en.wikipedia.org/wiki/Schist ''schist''], [https://en.wikipedia.org/wiki/Quartzite ''quartzite'']), | ||
+ | Most clastic sediments are terrigenous materials eroded from land. Erosion of rocky shores and submarine rock also produces clastic sediments. About 85% of all coastal marine sediments are clastic sediments<ref name=H/>. | ||
− | The products of rock weathering are transported mainly by rivers ( | + | The products of terrestrial rock weathering are transported mainly by rivers (present-time fluvial sediment supply is indicated in Fig. 1), but also by wind, ice and waves. The particles and fragments abrade each other when they are transported by the water flow and erode the surfaces over which they pass, thus contributing to the further breakdown of rock fragments. Time and distance are important factors: the longer the journey to the sea, the more chance there is for mineral grains to be rounded and reduced in size by abrasion. Downstream fining rates strongly differ between rivers<ref>Reyolds, T. 2024. Grain size from source to sink – modern and ancient fining rates. Earth-Science Reviews 250, 104699</ref>. During the downstream journey, grains are sorted according to density, size and shape, and chemically less stable minerals are dissolved. The most common solid products of weathering are rock fragments, quartz and clay minerals. Quartz is the only common rock mineral that is both hard (resistant to abrasion) and chemically stable at the Earth's surface. This is the reason why quartz is the predominant mineral in present day beach and river sands and is also common in most ancient sandstones. The rate at which weathering occurs depends on the local climate, with rapid breakdown in tropical areas, favoured by abundant rainfall and high temperatures, and with slow weathering occurring in deserts, where water is absent. In high-latitude zones, alternating frost and thaw and glacier dynamics are important weathering agents<ref name=H/>. |
Line 18: | Line 23: | ||
− | A minor fraction of terrigenous material is deposited from the atmosphere. | + | A minor fraction of terrigenous material is deposited from the atmosphere. It consists of dust carried by the wind and ash emitted by volcanoes and contains mainly very fine sand (quartz), but also silt and clay particles with significant amounts of aluminum (Al), iron (Fe), magnesium (Mg) and calcium (Ca ). Supply of atmospheric dust may be a minor factor in most areas today, except from major deserts, e.g., the Sahara, but in the past it has been substantial and has led to the accumulation of extensive loess deposits, e.g., in China. The exceptionally high sediment load of the Yellow river is due to erosion of these loess deposits, which are finally discharged into the Bohai Sea. |
− | In arctic and subarctic areas and where mountains are | + | In arctic and subarctic areas and where mountains are high enough so as to generate significant quantities of snowfall, glaciers produce substantial amounts of sediment with the meltwater flow through subglacial tunnels. |
− | Clastic sediments can be classified according to grainsize, see Table 1. Grainsizes are indicative of the advancement of the weathering process; small grains derive from weathering of larger grains. Sediment samples generally contain grains of different sizes. Sedimentary deposits are named after the largest present grains (clasts) | + | Clastic sediments can be classified according to grainsize, see Table 1. Grainsizes are indicative of the advancement of the weathering process; small grains derive from weathering of larger grains. Sediment samples generally contain grains of different sizes. Sedimentary deposits are named after the largest present grains (clasts). |
− | |||
− | |||
− | |||
− | |||
− | |||
− | |||
− | |||
− | |||
− | |||
− | |||
− | {| style="border-collapse:collapse;background:ivory;" cellpadding=5px align=center width= | + | {| style="border-collapse:collapse;background:ivory;" cellpadding=5px align=center width=85% |
− | |+ Table 1. Sedimentary particles, grainsize and the corresponding | + | |+ Table 1. Sedimentary particles, grainsize and the corresponding sedimentary rock type. The grainsize range is expressed in d[mm] and in <math>\phi=-log_2 d[mm]</math>. Adapted from (Hugget, 2007)<ref name=H></ref>. |
− | Adapted from (Hugget, 2007)<ref name=H></ref>. | ||
|- style="font-weight:bold; text-align:center; background:lightblue" | |- style="font-weight:bold; text-align:center; background:lightblue" | ||
! width="15% style=" border:1px solid blue;"| particle type | ! width="15% style=" border:1px solid blue;"| particle type | ||
! width="15% style=" border:1px solid bleu;"| particle name | ! width="15% style=" border:1px solid bleu;"| particle name | ||
− | ! width="15% style=" border:1px solid blue;"| grainsize [mm] | + | ! width="15% style=" border:1px solid blue;"| grainsize d[mm] |
− | ! width="25% style=" border:1px solid blue;"| | + | ! width="15% style=" border:1px solid blue;"| grainsize <math>\phi</math> |
+ | ! width="25% style=" border:1px solid blue;"| lithified | ||
|- | |- | ||
| style="border:2px solid lightblue;text-align:center" rowspan="4| Gravel | | style="border:2px solid lightblue;text-align:center" rowspan="4| Gravel | ||
| style="border:2px solid lightblue;text-align:center"| Boulders | | style="border:2px solid lightblue;text-align:center"| Boulders | ||
| style="border:2px solid lightblue;text-align:center"| > 256 | | style="border:2px solid lightblue;text-align:center"| > 256 | ||
+ | | style="border:2px solid lightblue;text-align:center"| < (-8) | ||
| style="border:2px solid lightblue;text-align:center" rowspan="4"| Conglomerate, breccia, | | style="border:2px solid lightblue;text-align:center" rowspan="4"| Conglomerate, breccia, | ||
− | gritstone, | + | gritstone, diamict |
|- | |- | ||
| style="border:2px solid lightblue;text-align:center"| Cobbles | | style="border:2px solid lightblue;text-align:center"| Cobbles | ||
| style="border:2px solid lightblue;text-align:center"| 64-256 | | style="border:2px solid lightblue;text-align:center"| 64-256 | ||
+ | | style="border:2px solid lightblue;text-align:center"| (-8)-(-6) | ||
|- | |- | ||
| style="border:2px solid lightblue;text-align:center"| Pebbles | | style="border:2px solid lightblue;text-align:center"| Pebbles | ||
| style="border:2px solid lightblue;text-align:center"| 4-64 | | style="border:2px solid lightblue;text-align:center"| 4-64 | ||
+ | | style="border:2px solid lightblue;text-align:center"| (-6)-(-2) | ||
|- | |- | ||
| style="border:2px solid lightblue;text-align:center"| Granules | | style="border:2px solid lightblue;text-align:center"| Granules | ||
| style="border:2px solid lightblue;text-align:center"| 2-4 | | style="border:2px solid lightblue;text-align:center"| 2-4 | ||
+ | | style="border:2px solid lightblue;text-align:center"| (-2)-(-1) | ||
|- | |- | ||
| style="border:2px solid lightblue;text-align:center" rowspan="5| Sand | | style="border:2px solid lightblue;text-align:center" rowspan="5| Sand | ||
| style="border:2px solid lightblue;text-align:center"| very coarse | | style="border:2px solid lightblue;text-align:center"| very coarse | ||
| style="border:2px solid lightblue;text-align:center"| 1-2 | | style="border:2px solid lightblue;text-align:center"| 1-2 | ||
+ | | style="border:2px solid lightblue;text-align:center"| (-1)-0 | ||
| style="border:2px solid lightblue;text-align:center" rowspan="5| Sandstone,arkose, | | style="border:2px solid lightblue;text-align:center" rowspan="5| Sandstone,arkose, | ||
greywacke, flags | greywacke, flags | ||
Line 67: | Line 67: | ||
| style="border:2px solid lightblue;text-align:center"| coarse | | style="border:2px solid lightblue;text-align:center"| coarse | ||
| style="border:2px solid lightblue;text-align:center"| 0.5-1 | | style="border:2px solid lightblue;text-align:center"| 0.5-1 | ||
+ | | style="border:2px solid lightblue;text-align:center"| 0-1 | ||
|- | |- | ||
| style="border:2px solid lightblue;text-align:center"| medium | | style="border:2px solid lightblue;text-align:center"| medium | ||
| style="border:2px solid lightblue;text-align:center"| 0.25-0.5 | | style="border:2px solid lightblue;text-align:center"| 0.25-0.5 | ||
+ | | style="border:2px solid lightblue;text-align:center"| 1-2 | ||
|- | |- | ||
| style="border:2px solid lightblue;text-align:center"| fine | | style="border:2px solid lightblue;text-align:center"| fine | ||
| style="border:2px solid lightblue;text-align:center"| 0.125-0.25 | | style="border:2px solid lightblue;text-align:center"| 0.125-0.25 | ||
+ | | style="border:2px solid lightblue;text-align:center"| 2-3 | ||
|- | |- | ||
| style="border:2px solid lightblue;text-align:center"| very fine | | style="border:2px solid lightblue;text-align:center"| very fine | ||
| style="border:2px solid lightblue;text-align:center"| 0.0625-0.125 | | style="border:2px solid lightblue;text-align:center"| 0.0625-0.125 | ||
+ | | style="border:2px solid lightblue;text-align:center"| 3-4 | ||
|- | |- | ||
| style="border:2px solid lightblue;text-align:center"| Silt | | style="border:2px solid lightblue;text-align:center"| Silt | ||
| style="border:2px solid lightblue;text-align:center"| | | style="border:2px solid lightblue;text-align:center"| | ||
| style="border:2px solid lightblue;text-align:center"| 0.002-0.0625 | | style="border:2px solid lightblue;text-align:center"| 0.002-0.0625 | ||
− | | style="border:2px solid lightblue;text-align:center" rowspan="2| Siltstone, | + | | style="border:2px solid lightblue;text-align:center"| 4-9 |
− | mudstone | + | | style="border:2px solid lightblue;text-align:center" rowspan="2| Siltstone, mudstone, marl |
|- | |- | ||
| style="border:2px solid lightblue;text-align:center"| Clay | | style="border:2px solid lightblue;text-align:center"| Clay | ||
| style="border:2px solid lightblue;text-align:center"| | | style="border:2px solid lightblue;text-align:center"| | ||
| style="border:2px solid lightblue;text-align:center"| < 0.002 | | style="border:2px solid lightblue;text-align:center"| < 0.002 | ||
+ | | style="border:2px solid lightblue;text-align:center"| > 9 | ||
|} | |} | ||
===Biogenic sediments <ref name=T> Taylor, K.G. 2008. Sediments and sedimentation. In: An Introduction to Physical Geography and the Environment (J. Holden, editor), Pearson Education Limited</ref>=== | ===Biogenic sediments <ref name=T> Taylor, K.G. 2008. Sediments and sedimentation. In: An Introduction to Physical Geography and the Environment (J. Holden, editor), Pearson Education Limited</ref>=== | ||
− | Most biogenic sediments are erosion products of unconsolidated or consolidated marine detrital deposits or erosion products of framework-building organisms (e.g. coral reefs). These sediments, called "bioclastic sediments", are composed of fragments of organic skeletal materials . They mainly consist of calcium carbonate (CaCO<sub>3</sub>) in the form of calcite crystals or aragonite crystals. Other biogenic sediments, such as dead plant remains (peat), have a high organic component and are termed ''organic-rich biological sediments''. Biogenic sediments may constitute a substantial fraction of the bed material in coastal environments where the supply of terrigenous material is small (no nearby river deltas). Many beaches in the tropics and subtropics consist almost entirely of carbonate sands which are derived from adjacent reefs composed of corals, skeletal material, shell fragments or precipitated calcium carbonate. Fine bioclastic sediments may also accumulate in sheltered coastal environments in temperate climate zones. An example is the inner part of the mega-tidal Baie du Mont Saint Michel (almost no fluvial sediment input), where tidal flats are composed of so-called ''tangue'' – low-cohesive fine sediment with a high biogenic content <ref> Desguée, R., Robin, N., Gluard, L., Monfort, O., Anthony, E.J. and Levoy, F., 2011. Contribution of hydrodynamic conditions during shallow water stages to the sediment balance on a tidal flat: Mont-Saint-Michel bay, Normandy, France. Estuarine, Coastal and Shelf Science, 94, 343-354</ref>. | + | Most biogenic sediments are erosion products of unconsolidated or consolidated marine detrital deposits or erosion products of framework-building organisms (e.g. coral reefs). These sediments, called "bioclastic sediments", are composed of fragments of organic skeletal materials . They mainly consist of calcium carbonate (CaCO<sub>3</sub>) in the form of [https://en.wikipedia.org/wiki/Calcite calcite crystals] or [https://en.wikipedia.org/wiki/Aragonite aragonite crystals]. Other biogenic sediments, such as dead plant remains (peat), have a high organic component and are termed ''organic-rich biological sediments''. Biogenic sediments may constitute a substantial fraction of the bed material in coastal environments where the supply of terrigenous material is small (no nearby river deltas). Many beaches in the tropics and subtropics consist almost entirely of carbonate sands which are derived from adjacent reefs composed of corals, skeletal material, shell fragments or precipitated calcium carbonate. Fine bioclastic sediments may also accumulate in sheltered coastal environments in temperate climate zones. An example is the inner part of the mega-tidal Baie du Mont Saint Michel (almost no fluvial sediment input), where tidal flats are composed of so-called ''tangue'' – low-cohesive fine sediment with a high biogenic content <ref> Desguée, R., Robin, N., Gluard, L., Monfort, O., Anthony, E.J. and Levoy, F., 2011. Contribution of hydrodynamic conditions during shallow water stages to the sediment balance on a tidal flat: Mont-Saint-Michel bay, Normandy, France. Estuarine, Coastal and Shelf Science, 94, 343-354</ref>. |
+ | |||
+ | Bioclastic sediments deposited in coastal waters can be lithified into limestone by so-called diagenesis. Thick deposits are cemented under pressure through dissolution of carbonate minerals and subsequent redeposition in the pore spaces. Limestone formation is a slow process that takes place on geological timescales. This contrasts with the decadal timescale of beachrock formation. Beachrock is formed in carbonate-rich nearshore (intertidal) waters, especially in the tropics or subtropics. Limestone fragments, skeletal fragments, quartz sand and other materials are cemented by carbonate precipitation, promoted by geochemical and microbial processes<ref>Vousdoukas, M.I., Velegrakis, A.F. and Plomaritis, T.A. 2007. Beachrock occurrence, characteristics, formation mechanisms and impacts. Earth-Science Reviews 85: 23–46</ref>. | ||
===Chemical sediments=== | ===Chemical sediments=== | ||
− | Chemical sediments form by precipitation of minerals out of solution as the water becomes saturated, which is often due to evaporation. The most common chemical sediments formed in this way are calcite (CaCO<sub>3</sub>), gypsum (CaSO<sub>4</sub>) and halite (NaCl). They are a common type of sediment of sabkhas, back-barrier coastal plains which are ubiquitous in arid climates. | + | Chemical sediments form by precipitation of minerals out of solution as the water becomes saturated, which is often due to evaporation. The most common chemical sediments formed in this way are calcite (CaCO<sub>3</sub>), gypsum (CaSO<sub>4</sub>.2H<sub>2</sub>O) and halite (NaCl). They are a common type of sediment of sabkhas, back-barrier coastal plains which are ubiquitous in arid climates. |
Line 139: | Line 146: | ||
|} | |} | ||
− | + | * [https://en.wikipedia.org/wiki/Feldspar Feldspars]: Aluminum silicates. The most common forms are: orthoclase KAlSi<sub>3</sub>O<sub>8</sub>, albite NaAlSi<sub>3</sub>O<sub>8</sub> and anorthite CaAl<sub>2</sub>Si<sub>2</sub>O<sub>8</sub>. These minerals are fairly hard, often with pink, white, or grey in color. About 40% of the lithosphere consists of feldspars. | |
− | * Feldspars: | ||
* Quartz: silicon dioxide crystal (SiO<sub>2</sub>); | * Quartz: silicon dioxide crystal (SiO<sub>2</sub>); | ||
− | * Mica: complex silicate minerals comprising elements K or Na and metal cations such as Al, Mg and Fe, that form crystals with a sheet-like arrangement of atoms; | + | * [https://en.wikipedia.org/wiki/Mica Mica]: complex silicate minerals comprising elements K or Na and metal cations such as Al, Mg and Fe, that form crystals with a sheet-like arrangement of atoms; |
− | * Clay: hydrous silicates that contain metal cations, generally | + | * Clay: hydrous silicates that contain metal cations, generally aluminum. Most common clay minerals are [https://en.wikipedia.org/wiki/Kaolinite kaolinite] (Al<sub>2</sub>Si<sub>2</sub>O<sub>5</sub>(OH)<sub>4</sub>), [https://en.wikipedia.org/wiki/Illite illite], [https://en.wikipedia.org/wiki/Smectite smectite] (incl. [https://en.wikipedia.org/wiki/Montmorillonite montmorillonite]), [https://en.wikipedia.org/wiki/Vermiculite vermiculite] and [https://en.wikipedia.org/wiki/Bentonite bentonite]. Their basic building blocks are sheets of silica (Si) tetrahedra and oxygen (O) and hydroxyl (OH) octahedra. Micas and clay minerals result originally from chemical weathering of igneous rocks and feldspars. For example, weathering of gabbro or basalt releases smectite, while weathering of granite or rhyolite releases illite<ref>Zhao, T., Xu, S. and Hao, F. 2023. Differential adsorption of clay minerals: Implications for organic matter enrichment. Earth-Science Reviews 246, 104598</ref>. Clay minerals are present in many sedimentary rocks, e.g., sandstone, greywacke, shale, mudstone, siltstone, and slate. |
− | The specific densities (density relative to water) of these minerals is given in Table 2. | + | The specific densities (density relative to water <math>s=\rho_{mineral}/\rho_{water}</math>) of these minerals is given in Table 2. |
Line 153: | Line 159: | ||
===Fall velocity=== | ===Fall velocity=== | ||
− | [[Image:FallVelocityQuartz.jpg|thumb|300px|left|Figure 3: Fall velocity of quartz spheres in still water.]] | + | [[Image:FallVelocityQuartz.jpg|thumb|300px|left|Figure 3: Fall velocity of quartz spheres (specific density <math>s=\rho/\rho_{water}=2.65</math>) in still water at temperature <math>\theta=20^{o}C</math>. The fall velocity at other temperatures <math>\theta</math> can be deduced by multiplying grainsizes <math>d[mm]</math> by <math>1-0.016*(\theta-20)</math>. ]] |
+ | |||
+ | |||
+ | The seabed of oceans and coastal waters are mainly formed by settling of sediment particles out of suspension. Which particles settle where is mainly determined by the particle fall velocity, concentration and turbulence. Under energetic conditions only coarse grades may settle. The fall velocity of sediment particles depends on size and density. Because the density of different types of sediments is similar (Table 2), the grain size is determinant. The larger the grain size, the higher the fall velocity. The fall velocity as a function of the grainsize is displayed in Fig. 3. Indicated is the fall velocity of round quartz grains in still water at low concentration. In reality, sediment particles do not have a round shape, which slightly affects the fall velocity. The actual fall velocity in flowing water is very different, due to up and down motions caused by turbulent fluid motions ('turbulent eddies'). The concentration of sediment particles also plays a role. At very high concentration, the fall velocity decreases, because particles increasingly interfere with each other, a phenomenon that is called hindered settling<ref>Winterwerp, J.C. 2002. On the flocculation and settling velocity of estuarine mud. Continental Shelf Research 22: 1339–1360</ref>. | ||
+ | <br clear=all> | ||
+ | ===Flocculation=== | ||
+ | |||
+ | [[Image:FlocRelativeFallVelocity.jpg|thumb|250px|left|Figure 4: The ratio of floc fall velocity and fall velocity of the constituent particles <ref > Migniot, C. 1968. A study of the physical properties of various forms of very fine sediments and their behaviour under hydrodynamic action. La Houille Blanche 7, 591–620</ref>.]] | ||
+ | |||
+ | If the fall velocity for very small particles, such as clay minerals, is derived from settling quartz spheres (Fig. 3), it seems so low that they will almost never reach the bottom from suspension. The ubiquitous mud beds in coastal waters point to a different settling mechanism. This mechanism consists of flocculation. Clay minerals have a large surface area relative to their weight, and electrical surface charges. This ensures that they easily bind to one another and to other substances in the water. Clays are therefore classified as ''cohesive sediments''. A substance that serves as a powerful binder is so-called EPS, [https://en.wikipedia.org/wiki/Extracellular_polymeric_substance extracellular polymeric substances] <ref> Grabowski, R.C., Droppo, I.G. and Wharton, G. 2011. Erodibility of cohesive sediment: the importance of sediment properties. Earth Science Reviews 105 (3-4): 101-12</ref>. These large organic molecules (polysaccharides, proteins, nucleic acids and lipids) are exuded by living organisms and therefore omnipresent in coastal waters. Flocs therefore grow much faster in natural seawater than in salinized distilled water<ref>Skinnebach, K.H., Fruergaard, M. and Andersen, T.J. 2019. Biological effects on flocculation of fine-grained suspended sediment in natural seawater. Estuarine, Coastal and Shelf Science 228, 106395</ref>. In addition to EPS, bacterial colonization also plays a role in flocculation <ref> Linley, E.A.S. and Field, J.G. 1982. The nature and significance of bacterial aggregation in a nearshore upwelling ecosystem. Estuarine, Coastal and Shelf Science 14: 1-11</ref>. Flocculation is further influenced by factors such as salinity and pH of the water. When flocs grow, they not only capture smaller flocs that settle more slowly, but also other suspended sediments, such as clay, silt and fine sand. Frequent encounters between sediment particles are important for floc growth. Flocculation is thus enhanced with a high concentration of suspended material and with a certain (low) degree of turbulence (often expressed as the turbulent shear rate <math>G</math>, see below), dependent on the suspended sediment concentration<ref>Mietta, F., Chassagne, C., Manning, A.J. and Winterwerp, J.C. 2009. Influence of shear rate, organic matter content, pH and salinity on mud flocculation. Ocean Dynamics 59: 751–763</ref>. However, when turbulence is strong (the general case in estuaries), large flocs are broken up. | ||
+ | |||
+ | Flocs settle much faster than the individual constituent particles - a factor of a thousand or more, see Fig. 4. The largest flocs are agglomerates of ''microflocs''. These so-called ''macroflocs'' (typical size > 160 <math>\mu</math>m) have the highest fall velocity, but they are less stable than the smaller microflocs (typical size < 160 <math>\mu</math>m). The most important factors that determine the fall velocity are the grainsize of the constituent particles, the grainsize of the constituent microflocs, the near-bed shear stress, the suspension concentration and the turbulent shear rate. | ||
+ | Further details on flocculation can be found in the article [[Flocculation cohesive sediments]]. | ||
+ | |||
+ | ===Sediment transport=== | ||
+ | The processes that underlie sediment transport are strongly related to turbulent flow structures in a thin boundary layer near the bed and above. Because these processes are very complicated and not even fully understood, empirical formulas are used in practice for the description of sediment transport. These formulas are described in the articles [[Sand transport]] and [[ Sediment transport formulas for the coastal environment]]. These articles mainly deal with non-cohesive sediments. Transport of cohesive sediments is dealt with in the articles [[Dynamics of mud transport]] and [[Sediment deposition and erosion processes]]. For measurement of sediment transport see: [[Measuring instruments for sediment transport]], [[Laboratory and in situ analysis of samples]]. | ||
− | + | ===Sediment deposits in coastal waters=== | |
+ | A more detailed introduction to sediment deposition and erosion is given in the article [[Sediment deposition and erosion processes]]. | ||
+ | Deposition of sediment on the seabed takes place when conditions are suitable. These conditions are different for each type of sediment. | ||
+ | When a sediment-laden water mass reaches an area of lower flow strength and wave activity, part of the carried material is deposited. Sediment particles with the greatest fall velocity settle first and particles entrained as bedload (rolling and jumping along the bottom) come to rest. When the current strength and wave activity further decrease, the fine suspended material also settles. In tidal environments, most sedimentation takes place in the period around [[slack water]] (flow reversal). Part of the deposited material will afterwards be resuspended by the recovering tidal flow, but another part will remain. This can lead to temporary or permanent deposition. Deposits are temporary if they are insufficiently consolidated and re-eroded during conditions of strong currents (e.g., spring tide) or strong wave action (storm). Permanent deposits have a layered character; each layer represents a deposition period. Successive layers may contain different types of sediment if they have been deposited under different conditions. The layered sediment deposits in estuarine channels sometimes exhibit thin intermediate mud drapes deposited in short periods around [[Slack water|high-water or low-water slack tide]]<ref name=MB>Martinius, A.W. and van den Berg, J.H. 2011. Atlas of sedimentary structures in estuarine and tidally influenced river deposits of the Rhine-Meuse-Scheldt system. EAGE Publ. ISBN 978-90-73834-11-8</ref>. | ||
+ | Anell (2024<ref>Anell, I. 2024. The quintessential s-shape in sedimentology: A review on the formation and controls of clinoform shape. Earth-Science Reviews 254, 104821</ref>) formulated the following empirical rules for inclined sedimentary deposits: | ||
+ | * Deposition occurs as the capacity to transport sediment declines | ||
+ | * Bedform height is generally ruled by accommodation (space available for deposition) and/or energy/agitation | ||
+ | * Smaller bedforms can advance faster to merge with larger bedforms | ||
+ | * Systems can build up to the [[angle of repose]] | ||
+ | * The [[angle of repose]] is often associated with the formation of linear slopes (or linear segments) | ||
+ | * Coarser grain size is associated with steeper slopes | ||
+ | * Higher mud content is associated with lower slope angles | ||
+ | * Increased pore pressure, associated with high sediment input, can generate failure at low slope angles, where muddy sediment fails at lower angles than sandy sediment | ||
+ | * Sandy slopes tend to be more curved than muddy slopes | ||
+ | * Curvature is an indication of contrast/change along the profile (lithology, grain size, deposition - or lack thereof - or type of depositional process) | ||
+ | * In larger bedforms, steepening with increasing depth is likely the effect of reduced 'relative' sedimentation with increasing accommodation | ||
+ | ===Graded sediment=== | ||
+ | [[Image:SedimentMixtures.jpg|thumb|400px|left|Figure 5: Classification of different types of graded sediment beds. Redrawn from Blair and McPherson (1999)<ref>Blair, T.C. and McPherson, J.G. 1999. Grain-size and textural classification of coarse sedimentary particles. Journal of Sedimentary Research 69: 6–19 </ref>.]] | ||
+ | Sediment deposits in coastal areas are not always layered; mixed deposits occur frequently. These deposits are referred to as graded sediment. Layered sediment deposits can be mixed when the seabed is strongly perturbated. Mixing also takes place through bioturbation: soil animals (mainly worms and molluscs) bring material from deeper layers to the surface through ingestion and excretion <ref>Baumfalk, Y.A. 1979. Heterogeneous grain size distribution in tidal flat sediment caused by bioturbation activity of Arenicola marina (polychaeta). Netherlands Journal of Sea Research 13: 428-440</ref><ref> Gallagher, E.D. 2008. Bioturbation. Biol. Ocean. Processes, EEOS 630</ref>. Deposition of mixed sediment also takes place by excretion of filter feeders in the form of fecal pellets. Terminology for mixed deposits is indicated in Fig. 5 for different mixtures of gravel, sand and mud. Mud is itself a mixture of particles with a grain size of less than 0.063 mm, consisting of very fine sand, silt, clay and organic matter. | ||
+ | |||
+ | See also: [[Biogeomorphology of coastal systems]], [[Sandy shore habitat]], [[Meiofauna of Sandy Beaches]]. | ||
+ | |||
+ | ====Vertical segregation, bed armoring==== | ||
+ | A graded sediment bed stirred by an oscillatory current can undergo inverse grading by a process called 'kinematic vertical sorting', see [[Seabed armoring]]. Coarse sediments are lifted gradually to the seabed surface, leading to the formation of a coarse armor top layer that covers a sublayer of finer sediment. These finer sediments are therefore better protected from erosion. | ||
+ | |||
+ | ====Horizontal segregation==== | ||
+ | Spontaneous horizontal segregation of graded sediment can occur under certain conditions. This segregation generates contiguous patches of different types of sediment. The underlying feedback mechanism links sedimentation of fine material on a smooth muddy seabed to a locally reduced degree of turbulence <ref> Murray, A.B. and Thieler, E.R. 2004. A new hypothesis and exploratory model for the formation of large-scale inner-shelf sediment sorting and ‘rippled scour depressions’. Continental Shelf Res. 24: 295-315</ref>. As a result, fine material will mainly deposit in places where fine material already dominates on the seabed, so that these muddy patches increase in size and in mud content. This continues until no fine material is available any more from neighboring patches of coarser deposits from which the fines have been winnowed. | ||
+ | |||
+ | ===Bedforms=== | ||
+ | [[Image:MigratingDuneStructure.jpg|thumb|350px|right|Figure 6: Cross-stratification of a migrating dune. Top: Migrating dune in unidirectional flow. The layer inclination follows the leeside slope; this is called form-concordant. Bottom: Migrating dune in alternating flow (wave orbital flow, tidal flow) with dominant flow direction. The stratification at the stoss side of the dune follows the leeside dune slope of the previous opposite-flow period; this is called form-discordant. Laminae correspond to episodic avalanching events along the slip face. The slip face angle <math>\alpha</math> is close to the subaqueous angle of repose of the sediment. The discontinuity plane corresponds to the partially eroded surface of a previous bedform. The laminae and discontinuity planes are preserved in the stratigraphic record. Adapted from <ref>Reineck, H.-E. and Singh, I.B. 1973. Depositional sedimentary environments. Springer, Berlin, 439 pp.</ref> ]] | ||
− | = | + | Sediment layers are generally horizontal, but can also have a wavy character. These undulations are caused by the fact that the interaction between flow and sediment bed does not behave linearly, meaning that small disturbances of the flat sediment bed can start growing exponentially. This leads to the emergence of a large range of bedforms, as explained in the article [[Stability models]]. The smallest bedforms, ripples, arise in places where bed sediments are sandy and where currents and wave activity are not very strong but sufficient to set sediment in motion. This is explained in the articles [[Wave ripples]] and [[Wave ripple formation]], for situations where sediment movement is mainly determined by waves. When wave activity increases, while the background current remains small, ripples can transform into patchy seabed structures called hummocks <ref name=BN>Van den Berg, J.H. and Nio, S.D. 2010. Sedimentary structures and their relation to bedforms and flow conditions. EAGE Publications</ref>. Bed ripples exert friction on tidal currents and have a great influence on the flow velocity. More details can be found in the articles [[Bedforms and roughness]] and [[Bed roughness and friction factors in estuaries]]. In addition to ripples, much larger bedforms can also arise, for example: megaripples (wave length of several meters), transverse and longshore bars (wave length of the order of one hundred meters, see [[Rhythmic shoreline features]]), dunes (also called sandwaves, wavelength of tens to hundreds of meters), [[chenier]]s (sand ridges on muddy shorelines, wavelength of the order of one hundred meters<ref>Augustinus, P.G.E.F. 1989. Cheniers and chenier plains: a general introduction. Marine Geology 90: 219-229</ref>) and shoreface-connected ridges and tidal ridges (wavelength of several kilometers, see [[Sand ridges in shelf seas]]). Different bedforms scales mentioned may be found superimposed on each other. In the underlying sedimentary layers, bed ripples are not always preserved, but the larger bedforms can generally be observed quite well. |
− | + | The typical layer structure of the seabed that occurs under the stoss slope of migrating bedforms, such as (mega)ripples or dunes, is called cross-stratification. The layer structure consists of parallel laminae formed by sediment avalanching at the migrating bedform crest (more precisely, at the brinkpoint, sligtly downstream of the crest). The laminae are strongly dipping in the direction of bedform migration, according to the angle repose, see Fig. 6. | |
− | + | The bedforms described above do not occur with deposits of fine cohesive material (mud). Dewatering of freshly deposited mud is a slow process and mud layers therefore remain fluid for a long time as so-called [[Definitions of coastal terms#Mud|fluid mud]], see for example the article [[Dynamics of mud transport]]. Once consolidated, the erosion resistance is high, so that no bed ripples can form. Mud layers therefore have a smooth surface and exert little friction on the flow. | |
− | |||
− | + | ===Large-scale sediment sorting=== | |
+ | Sediment sorting, the spatial segregation of different types of sediment deposits, is a characteristic of mobile sediment beds. As indicated earlier, sediment deposition requires suitable hydrodynamic conditions, which depend on the type of sediment. Fine sediments cannot settle in high-energy environments (strong waves, strong currents). Temporary settling is possible when currents are weak (neap tide, slack tide) and in the absence of strong wave action. However, in situations where low-energy and high-energy conditions alternate, these temporary deposits will disappear. Although currents generally carry a mixture of different types of sediment, remaining deposits will only comprise grainsizes that cannot be resuspended under the most energetic conditions among the alternating conditions that occur at a specific location. Grain size analysis of sediment deposits therefore can provide an indication of the maximum hydrodynamic shear stresses that occur at a particular location<ref>Ward, S.L., Neill, S.P., Van Landeghem, K.J.J. and Scourse, J.D. 2015. Classifying seabed sediment type using simulated tidal-induced bed shear stress. Marine Geology 367: 94–104</ref><ref>Escobara, C.A., Mayerle, R. and Restrepo, D. 2019. Estimation of sediment grain sizes in a mesotidal area, Dithmarschen Bight, German North Sea. Marine Geology 417, 106006</ref>. A qualitative overview of the types of sediment deposits that form the seabed top layer under different hydrodynamic conditions is indicated in Table 3 for sandy coastal environments, together with the associated sediment transport modes and bedforms. These bedforms are themselves cause of sediment sorting. In low-energetic environments (weak currents, waves) the coarsest sediments accumulate in the troughs (swales) by preferential downward motion along the slip face of the bedforms<ref name=BN></ref>. In the case of stronger waves and currents the coarsest sediments are found at the bedform crest where fine sediments are most easily brought in suspension <ref>Van Oyen, T., Blondeaux, P. and Van den Eynde, D. 2013. Sediment sorting along tidal sand waves: A comparison between field observations and theoretical predictions. Continental Shelf Research 63: 23–33</ref>. | ||
− | |||
− | + | {| style="border-collapse:collapse;background:ivory; font-size: 14px " cellpadding=5px align=center width=100% | |
+ | |+ Table 3. Sediment sorting in sandy coastal environments: correspondence between hydrodynamic conditions (highest energy conditions among the usual alternating tidal, climatic and seasonal conditions) and seabed deposit; <math>U_c</math> is the maximum depth-averaged tidal current velocity and <math>U_w</math> is the wave-orbital velocity at the seabed. The associated bedforms and sediment transport modes are also indicated. The table is adapted from Terwindt (1981) <ref name=Ter>Terwindt, J.H.J. 1981. Origin and sequences of sedimentary structures in inshore mesotidal deposits of the North Sea. Spec. Publs. Int. Ass. Sediment. 5: 4-26</ref> and Martinius and van den Berg (2011)<ref name=MB></ref>. | ||
+ | |- style="font-weight:bold; font-size: 12px; text-align:center; background:lightblue" | ||
+ | ! width="30% style=" border:1px solid blue; font-size: 12px;"| Hydrodynamic conditions | ||
+ | ! width="25% style=" border:1px solid bleu; font-size: 12px;"| Sediment | ||
+ | ! width="25% style=" border:1px solid blue; font-size: 12px;"| Bedform | ||
+ | ! width="20% style=" border:1px solid blue; font-size: 12px;"| Sediment transport mode | ||
+ | |- | ||
+ | | style="border:2px solid lightblue; font-size: 12px; text-align:center"| Strong currents <math>\small U_c > 1 m/s</math> | ||
+ | | style="border:2px solid lightblue; font-size: 12px;text-align:center"| Medium-coarse sand | ||
+ | | style="border:2px solid lightblue; font-size: 12px;text-align:center"| 3D dunes | ||
+ | | style="border:2px solid lightblue; font-size: 12px;text-align:center"| Suspended load | ||
+ | |- | ||
+ | | style="border:2px solid lightblue; font-size: 12px;text-align:center"| Medium currents <math>\small 0.5<U_c< 1 m/s</math> | ||
+ | | style="border:2px solid lightblue; font-size: 12px;text-align:center"| Fine-medium sand, mud drapes | ||
+ | | style="border:2px solid lightblue; font-size: 12px;text-align:center"| Ripples, 2D dunes | ||
+ | | style="border:2px solid lightblue; font-size: 12px;text-align:center"| [[Definitions of coastal terms#Bedload|Bedload]], suspended load | ||
+ | |- | ||
+ | | style="border:2px solid lightblue; font-size: 12px;text-align:center"| Weak currents <math>\small U_c< 0.5 m/s</math> | ||
+ | | style="border:2px solid lightblue; font-size: 12px;text-align:center"| Mud, fine sand | ||
+ | | style="border:2px solid lightblue; font-size: 12px;text-align:center"| Flat bed (mud), ripples (fine sand) | ||
+ | | style="border:2px solid lightblue; font-size: 12px;text-align:center"| Suspended load (mud), bedload (fine sand) | ||
+ | |- | ||
+ | | style="border:2px solid lightblue; font-size: 12px;text-align:center"| Strong waves <math>\small U_w>1 m/s</math> | ||
+ | | style="border:2px solid lightblue; font-size: 12px;text-align:center"| Fine-coarse sand | ||
+ | | style="border:2px solid lightblue; font-size: 12px;text-align:center"| Flat bed | ||
+ | | style="border:2px solid lightblue; font-size: 12px;text-align:center"| Suspended load, [[Definitions of coastal terms#Sheet-flow|sheet flow]] | ||
+ | |- | ||
+ | | style="border:2px solid lightblue; font-size: 12px;text-align:center"| Medium waves <math>\small 0.3<U_w<1 m/s</math> | ||
+ | | style="border:2px solid lightblue; font-size: 12px;text-align:center"| Fine-medium sand, mud drapes | ||
+ | | style="border:2px solid lightblue; font-size: 12px;text-align:center"| Wave ripples | ||
+ | | style="border:2px solid lightblue; font-size: 12px;text-align:center"| Bedload, suspended load | ||
+ | |- | ||
+ | | style="border:2px solid lightblue; font-size: 12px;text-align:center"| Weak waves, currents <math>\small U_w<0.3 m/s, U_c<0.5 m/s</math> | ||
+ | | style="border:2px solid lightblue; font-size: 12px;text-align:center"| Fine sand, mud layers | ||
+ | | style="border:2px solid lightblue; font-size: 12px;text-align:center"| Wave ripples (fine sand), flat bed (mud) | ||
+ | | style="border:2px solid lightblue; font-size: 12px;text-align:center"| Bedload, suspended load | ||
+ | |- | ||
+ | | style="border:2px solid lightblue; font-size: 12px;text-align:center"| Strong currents, strong waves <math>\small U_c, U_w>1 m/s</math> | ||
+ | | style="border:2px solid lightblue; font-size: 12px;text-align:center"| medium-coarse sand | ||
+ | | style="border:2px solid lightblue; font-size: 12px;text-align:center"| Flat bed | ||
+ | | style="border:2px solid lightblue; font-size: 12px;text-align:center"| Suspended load, sheet flow | ||
+ | |} | ||
− | |||
− | |||
− | |||
− | |||
− | |||
+ | ===Seabed erosion=== | ||
+ | When exposed to turbulent shear stresses due to currents or waves, sediment particles are dislodged from the seabed and lifted one by one or in lumps. For this, the shear stress must exceed a certain critical value, <math>\tau_{cr}</math>, which depends on many factors, in particular the grainsize (see Fig. 7). The white gap in Fig. 7 shows that seabed erosion is influenced by other factors than shear stress and grain size. These factors are<ref>Yang, Y., Gao, S., Wang, Y.P., Jia, J., Xiong, J. and Zhou, L. 2019. Revisiting the problem of sediment motion threshold. Continental Shelf Research 187, 103960</ref>: | ||
+ | [[File:ErosionShearStress.jpg |thumb|left|400px|Fig. 7. Seabed erosion or no erosion depending on shear stress as a function of the median sediment grainsize <math>d_{50}</math>. The white zone represents the influence on seabed erosion of various factors other than shear stress and grain size. [Pa]=[Nm<sup>-2</sup>]=[kg m<sup>-1</sup>s<sup>-2</sup>].]] | ||
− | + | * Specific density. The sediment specific density is not considered in this figure, because it is rather similar for most mineral sediments. This does not apply to sediments of biotic origin, in which case besides density, biochemical bonds also strongly affect erodibility. | |
− | + | * Angularity of sediment particles. Rounded grains are less susceptible to uptake than angular grains<ref> Paphitis, D. 2001. Sediment movement under unidirectional flows: an assessment of empirical threshold curves. Coastal Engineering. 43: 227-245</ref>. | |
+ | * Sediment sorting. When sediment deposits are a mixture of coarse and fine sediment, the surface layer will mainly consist of coarse particles that protect the underlying fine sediment from erosion (so-called bed armoring effect). However, the coarse grains will be more exposed and are therefore more easily expelled from the sediment bed. | ||
+ | * Peak turbulent intensity. The shear stress represents the average turbulent intensity. However, seabed erosion is sensitive to incidental peaks of high turbulent intensity (associated with turbulent bursting events). Some seabed erosion therefore can occur already at quite low values of the shear stress<ref>Mohtar, W.H.M.W., Lee, J.W., Azha, N.I.M. and Cheng, N-S. 2020. Incipient sediment motion based on turbulent fluctuations. International Journal of Sediment Research 35: 125-133</ref>. | ||
+ | * Consolidation. Fine sediment can be easily resuspended when it has just been deposited. Freshly deposited fine sediment has a large pore volume filled with seawater that can be set in motion under the influence of horizontal pressure gradients of the surface water. Over time, the seawater is forced out of the pores by the weight of the consolidating deposit. The bulk density of the deposit increases while the permeability decreases. This causes a sharp increase of the critical shear stress for erosion<ref name=M21>Mohr, H., Draper, S., White, D.J. and Cheng, L. 2021. The effect of permeability on the erosion threshold of fine-grained sediments. Coastal Engineering 163, 103813</ref>. | ||
+ | * Clay content of the deposit. The molecular structure of clay particles enables the formation of electrochemical bonds that significantly increase the resistance of the deposit against erosion. This effect already occurs at a clay content of just 10%. A deposit with a sufficiently high clay content retains the water for a long time and consolidates slowly. Once consolidated, the deposit forms a hard layer that can withstand very high shear stresses. See [[Sediment deposition and erosion processes]] for further details. | ||
+ | * Biota. Living organisms can strongly alter the erosion strength of seabed deposits. Various large organic molecules, called EPS (extracellular polymeric substances), secreted by micro-organisms, bind sediment particles together and form biofilms at the sediment surface, especially in intertidal areas. Erosion resistance of sediment deposits is also favored by the formation of microbial mats, often called algal mats. Erosion resistance is diminished by other organisms, bivalves in particular, that disturb the sediment bed by so-called bioturbation and graze on microalgae. More details can be found in [[Biogeomorphology of coastal systems]]. | ||
− | + | The amount of sediment <math>E</math> [kg s<sup>-1</sup>m<sup>-2</sup>] that is brought in suspension when the shear stress <math>\tau</math> exceeds the critical stress <math>\tau_{cr}</math> for initiation of erosion, primarily depends on the excess shear stress <math>\tau - \tau_{cr}</math>. However, it also depends on many other factors. For fine non-cohesive sand, the relation can be represented by the empirical formula<ref> Mehta, A. J. and Partheniades, E. 1982. Resuspension of deposited cohesive sediment beds. Procs. 18th International Conference on Coastal Engineering, Cape Town, South Africa</ref> | |
− | + | <math>E = M \, (\Large\frac{\tau}{\tau_{cr}}\normalsize -1)^n ,</math> | |
− | + | with <math>n \sim </math> 1 and <math>M \sim </math> 0.05-0.1 kg m<sup>-2</sup>s<sup>-1</sup>. For fine cohesive sediment, <math>M</math> and <math>n</math> strongly depend on the degree of consolidation and must be determined experimentally. The values found for <math>n</math> are generally much larger than 1 and the values of <math>M</math> much smaller than 0.1 kg m<sup>-2</sup>s<sup>-1</sup>, see [[Sediment deposition and erosion processes]] for further details. In practice, the coefficients <math>M</math> and <math>n</math> change with time because the consolidation degree of the seabed changes with depth. | |
− | |||
− | |||
− | === | + | ===Sediment contamination and bioavailability=== |
− | + | As indicated earlier, fine sediments - clay particles in particular - can easily bind with other substances in the water. This binding is called sorption: adsorption (=surface binding) or absorption (=uptake). This property allows fine sediments to filter out dissolved contaminants from the water. The water becomes less polluted, but the contamination of bed sediments, on the other hand, increases. The partition of pollutants between the dissolved phase and the sediment-bound phase is represented by the parameter <math> K_d </math>. This partition, i.e. the value of <math> K_d</math>, depends on the type of pollutant and the type of sediment. | |
− | |||
− | + | Laboratory tests show that for inorganic contaminants, for example heavy metals such as lead (cation Pb<sup>2 +</sup>), cadmium (Cd<sup>2 +</sup>) and copper (Cu<sup>2 +</sup>), the partition depends on the sediment grainsize <ref> Schorer, M. 1997. Pollutant and organic matter content in particle size fractions. Freshwater contamination, IAHS publ. 243.</ref>. The smaller the grainsize (i.e. greater surface/volume), the stronger is the sorption (large <math> K_d </math>), almost independently of the concentration of dissolved heavy metals. | |
− | + | Biogeochemical processes determine the degree to which trace metals (especially cadmium and copper) are bound to sediment, depending on several factors, such as salinity and oxygen content. In an estuary, the ratio between the bound and labile (bioavailable) fraction of trace metals is variable in space and time. For example, measurements in the Scheldt estuary show that the labile fraction is greatest in the salt-fresh transition zone<ref>Gaulier, C., Zhou, C., Gao, Y., Guo, W., Reichstädter, M., Ma, T. Baeyens, W. and Billon, G. 2021. Investigation on trace metal speciation and distribution in the Scheldt estuary. Science of the Total Environment 757, 143827</ref>. | |
− | |||
− | |||
− | + | For organic pollutants such as [[PCB]] s (polychlorinated biphenyls) and [[PAH]] s (polyciclic aromatic hydrocarbons), sorption to sediment is primarily determined by the organic carbon content in the sediment, rather than by the grain size<ref> Karickhoff, S.W., Brown, D.S. and Scott, T.A. 1979. Sorption of hydrophobic pollutants on natural sediments. Water Research 13: 241-248</ref>. This is a well-known feature in water sanitation technology, where active carbon is used to filter contaminants from waste water. | |
− | |||
− | Contaminants are much less toxic to marine organisms when they are bound to sediment than when they are dissolved in water <ref> National Research Council. 2003. Bioavailability of Contaminants in Soils and Sediments: Processes, Tools, and Applications. Washington, DC: The National Academies Press. doi: 10.17226/10523</ref>. The bioavailability | + | Contaminants are much less toxic to marine organisms when they are bound to sediment than when they are dissolved in water <ref name=NRC>National Research Council. 2003. Bioavailability of Contaminants in Soils and Sediments: Processes, Tools, and Applications. Washington, DC: The National Academies Press. doi: 10.17226/10523</ref>. The bioavailability decreases further as the sediment bed gets older. Perturbation of the sediment bed by dredging or by bioturbation, however, plays a role. When sediment is worked up from a deeper anoxic layer to a higher oxic layer, attached metals are released by desorption. Burial of sediments to deeper soil layers, on the other hand, reduces bioavailability. The bioavailability of contaminants in soils can be reduced by adding active carbon<ref name=NRC></ref>. |
Line 214: | Line 302: | ||
===Oceans=== | ===Oceans=== | ||
− | The deep oceans have four main sediment sources <ref name=T></ref>: | + | [[File:SeafloorSediment.png|thumb|400px|right|Fig. 8. Distribution of sediment types on the seafloor. From [https://en.wikibooks.org/wiki/Historical_Geology/Marine_sediments Wikibooks].]] |
− | # Clastic fluvial sediments. Large quantities of fine sediments (silt, clay and fine sand) are deposited by the world's major rivers at the edges of the continental shelfs | + | The deep oceans have four main sediment sources <ref name=T></ref> (Fig. 8): |
− | # Atmospheric dust. A large part of the fine sediments entrained by wind from arid land areas is deposited in the oceans (e.g., red clay deposits, manganese nodules). This contribution to ocean sedimentation is relatively small nowadays. | + | # Clastic fluvial sediments. Large quantities of fine sediments (silt, clay and fine sand) are deposited by the world's major rivers at the edges of the continental shelfs. These deposits destabilize the continental shelf slope, causing the sediment to slide down to the ocean floor by slumping or through gravity currents. These deposits are confined along the shelf break and are not further spread over the ocean floor. |
+ | # Atmospheric dust. A large part of the fine sediments entrained by wind from arid land areas and ash from volcanic eruption plumes is deposited in the oceans (e.g., red clay deposits, manganese nodules). This contribution to ocean sedimentation is relatively small nowadays. | ||
# Carbonaceous ooze. The remains of calcareous algae, mainly coccoliths and foraminifera, are a major component of the seafloor sediment in large parts of the ocean. Below depths of 3 to 4 km, however, they do not occur, because the scales dissolve here as a result of too high a pressure and too high a concentration of carbon dioxide. | # Carbonaceous ooze. The remains of calcareous algae, mainly coccoliths and foraminifera, are a major component of the seafloor sediment in large parts of the ocean. Below depths of 3 to 4 km, however, they do not occur, because the scales dissolve here as a result of too high a pressure and too high a concentration of carbon dioxide. | ||
# Siliceous ooze. The remains of silicate plankton, mainly diatoms and radiolaria, dominate in other parts of the ocean - especially in the central part of the Pacific and in the Antarctic Ocean. | # Siliceous ooze. The remains of silicate plankton, mainly diatoms and radiolaria, dominate in other parts of the ocean - especially in the central part of the Pacific and in the Antarctic Ocean. | ||
Line 222: | Line 311: | ||
===Beach and foreshore=== | ===Beach and foreshore=== | ||
− | [[Image:GrainsizeBeachslope.jpg|thumb|400px|left|Figure | + | [[Image:GrainsizeBeachslope.jpg|thumb|400px|left|Figure 9: Relation between fall velocity (sediment type) and beach slope as a function of the Dean parameter <math>\Omega</math>. Redrawn after (Flemming and Fricke, 1983)<ref>Flemming, B.W. and Fricke, A.H. 1983. Beach and nearshore habitats as a function of internal geometry, primary sedimentary structures and grain size. In: McLachlan, A., Ersamus, T. (Eds.), Sandy Beaches as Ecosystems. Dr. W. Junk Publishers, The Hague, pp. 115–132</ref>.]] |
− | Sediments on the foreshore and subaerial beach are derived from nearby rivers or from ancient offshore river deposits. The foreshore is a highly energetic environment due to the activity of waves, which prevent settling of fine sediments. Sand and gravel are the dominant seabed sediments, depending on the supply of these sediments. The coarsest sediments are found in the | + | Sediments on the foreshore and subaerial beach are derived from nearby rivers or from ancient offshore river deposits. The foreshore is a highly energetic environment due to the activity of waves, which prevent settling of fine sediments. Sand and gravel are the dominant seabed sediments, depending on the supply of these sediments. The coarsest sediments are found in the breaker zone, especially in the higher part where the waves collapse on the beach. The finer fractions are found in the offshore zone and also on the backshore and the dunes, which are fed from the dry beach by aeolian transport. The type of sediment on the shoreface largely determines the equilibrium shoreface slope for a given wave climate. The relationship between sediment grain size, wave climate and beach slope can be characterized by the Dean parameter |
<math>\Omega = H / (w T) , </math> | <math>\Omega = H / (w T) , </math> | ||
− | where <math>H</math> is the significant offshore wave height (before breaking), <math>w</math> the sediment fall velocity and <math>T</math> the peak wave period. This relationship is schematically shown in Fig. | + | where <math>H</math> is the significant offshore wave height (before breaking), <math>w</math> the sediment fall velocity and <math>T</math> the peak wave period. This relationship is schematically shown in Fig. 9, which holds for sandy or gravelly coasts. |
+ | [[Image:BeachSlopeGrainSizeData.jpg|thumb|400px|right|Figure 10: Correlation between sediment grainsize and related beach slope from 78 field studies, adapted from Bujan et al. (2019)<ref name=Bu>Bujan, N., Cox, R. and Masselink, G. 2019. From fine sand to boulders: Examining the relationship between beach-face slope and sediment size. Marine Geology 417, 106012</ref>. The violet area indicates the scatter of the data points.]] | ||
+ | Figure 9 suggests a positive correlation between sediment grainsize and beach slope (the beach slope is defined here as the average slope of the beach face, the zone between the low-water line and the beach berm). This is substantiated by a study of Bujan et al. (2019)<ref name=Bu></ref>, who compiled data on grainsize and associated beach slope (<math>\beta</math>) from a large number of field sites. The result is shown in Fig. 10, where the violet area represents more than 95% of the data points. The dark blue line is a fit to the observed values represented by the empirical relation <math>\tan \beta = -0.154(D_{50}-0.125)^{-0.145} + 0.268</math>. The large bandwidth around this line reflects the different characteristics of the field sites (wave climate, tides, beach profile, sediment sorting, ...) and the different data collection and analysis methods used in the underlying studies. Grainsize and beach slope are positively correlated. For large grainsizes, [[Swash zone dynamics|wave up- and downrush]] is reduced by infiltration into the the sediment bed. This is a plausible explanation for the weak dependence of beach slope on grainsize for coarse sediment, compared to the strong dependence for fine-medium sediment<ref name=Bu></ref>. | ||
+ | |||
+ | For a more detailed discussion of the shoreface equilibrium profile the reader is referred to the article [[Shoreface profile]], which deals with sandy coasts. | ||
+ | |||
+ | Coasts with abundant fine sediment supply are not well represented by the Dean parameter and the grainsize-beach slope relationship of Fig. 10. Large deposits of fine sediments can occur on the foreshore and upper shoreface of such coasts. These deposits absorb most of the energy of incident waves into a fluid mud layer which is maintained by absorption of wave energy. A more detailed description is given in the article [[Coastal mud belt]]. <br clear=all> | ||
===Estuaries=== | ===Estuaries=== | ||
− | The sediment distribution in estuaries is particularly complex. Sediment deposits depend on the supply of river sediment, the supply of sediment from the sea and on local flow and wave conditions, in relation with a generally intricate topography. In addition, deposits are influenced by strong fluctuations in river discharge, tides and wave conditions at various timescales. Nevertheless, the sediment distribution in estuaries generally displays a characteristic pattern that is shown in Fig. | + | The sediment distribution in estuaries is particularly complex. Sediment deposits depend on the supply of river sediment, the supply of sediment from the sea and on local flow and wave conditions, in relation with a generally intricate topography. In addition, deposits are influenced by strong fluctuations in river discharge, tides and wave conditions at various timescales. Nevertheless, the sediment distribution in estuaries generally displays a characteristic pattern that is shown in Fig. 11 and discussed below. |
− | [[Image:EstuarineBedSedimentDistribution.jpg|thumb|700px|center|Figure | + | [[Image:EstuarineBedSedimentDistribution.jpg|thumb|700px|center|Figure 11: Typical example of the spatial distribution of bed sediments in a coastal plain estuary, schematically represented (not at scale). Adapted from Dalrymple et al. (1992)<ref>Dalrymple, R.W., Zaitlin, B.A., Boyd, R., 1992. Estuarine facies models: conceptual basis and stratigraphic implications. Journal of Sedimentary Petrology 62, 1130–1146 </ref>.]] |
− | Gravel transported by rivers is mostly deposited upstream and does not reach coastal plain estuaries; rivers discharge mainly | + | Gravel transported by rivers is mostly deposited upstream and does not reach coastal plain estuaries. This is the case when catchment areas are situated far from the sea; long rivers discharge mainly sandy and muddy sediments into broad coastal plain estuaries. In the case of mountainous coasts, estuaries are generally small drowned river valleys (rias); they may receive substantial amounts of gravely sediments, which are further discharged to the coastal zone by strong river floods. Most coastal plain estuaries also receive sand and mud imported from the sea by tidal flood currents. The estuarine morphology is in morphodynamic equilibrium if, averaged over a long period, the inflow of sand and mud is balanced by export to the sea by outflowing ebb currents<ref>Dronkers, J. 2017. Convergence of estuarine channels. Cont. Shelf Res. 144: 120-133</ref>, see [[Morphology of estuaries]]. This is not a static equilibrium, because sand and mud are moving across the estuary all the time, including temporal deposition and erosion. |
− | Morphodynamic equilibrium does not imply that the concentration of suspended sediment in an estuary is uniform. Concentration maxima occur in convergence zones where temporal settling of sediments takes place. These convergence zones depend not only on flow strength and flow pattern, but also on transport, settling and erosion characteristics of sediment particles. The areas of highest suspended concentration and deposition thus differ for sand and mud <ref>Dronkers, J. 2017. Dynamics of coastal systems. World Scientific Publ. Co., 753 pp.</ref>. | + | Morphodynamic equilibrium does not imply that the concentration of suspended sediment in an estuary is uniform. Concentration maxima occur in convergence zones where temporal settling of sediments takes place (or ongoing settling in the case of non-equilibrium). These convergence zones depend not only on flow strength and flow pattern, but also on transport, settling and erosion characteristics of sediment particles. The areas of highest suspended concentration and deposition thus differ for sand and mud <ref>Dronkers, J. 2017. Dynamics of coastal systems. World Scientific Publ. Co., 753 pp.</ref>. |
Convergence of sand transport takes place on the outer delta, where the ebb flow expands and decelerates. Within the estuary, convergence zones of sand transport are marked by sand banks and sand bars which have grown at the junctions of ebb and flood flow channels and at the inner bends of flow meanders. | Convergence of sand transport takes place on the outer delta, where the ebb flow expands and decelerates. Within the estuary, convergence zones of sand transport are marked by sand banks and sand bars which have grown at the junctions of ebb and flood flow channels and at the inner bends of flow meanders. | ||
− | Convergence of mud transport (silt and clay) occurs in the transition zone between flood dominant near-bed flow (due to tidal asymmetry and estuarine density-driven circulation) and ebb dominant flow ( | + | Convergence of mud transport (silt and clay) occurs in the transition zone between flood dominant near-bed flow (due to [[Tidal asymmetry and tidal basin morphodynamics|tidal asymmetry]] and [[Estuarine circulation|estuarine density-driven circulation]]) and ebb dominant flow (river flow enhanced by ebb tidal currents). This transition zone has the highest mud concentrations, both in the water column (turbidity maximum) and in the channel bed (for more details, see the articles [[Estuarine turbidity maximum]], [[Dynamics of mud transport]], [[Sediment deposition and erosion processes]]). Besides, mud concentrations are high in sheltered areas where fine sediments can settle without immediately being resuspended, such as salt marshes and mangroves. In these areas, waves and currents are strongly reduced by vegetation, which enhances sediment deposition. See also: [[Dynamics, threats and management of salt marshes]] and [[Mangroves]]. |
+ | |||
+ | |||
+ | ==Related articles== | ||
+ | :[[Sediment deposition and erosion processes]] | ||
+ | :[[Dynamics of mud transport]] | ||
+ | :[[Shoreface profile]] | ||
+ | :[[Estuarine turbidity maximum]] | ||
+ | :[[Coastal mud belt]] | ||
+ | :[[ Wave-induced soil liquefaction]] | ||
==References== | ==References== | ||
− | |||
<references/> | <references/> | ||
− | {{ | + | {{2Authors |
− | | | + | |AuthorID1=120 |
− | | | + | |AuthorFullName1=Job Dronkers |
− | | | + | |AuthorName1=Dronkers J |
+ | |AuthorID2=39083 | ||
+ | |AuthorFullName2= Janrik van den Berg | ||
+ | |AuthorName2= Van den Berg J H | ||
+ | }} | ||
− | [[Category: | + | [[Category:Physical coastal and marine processes]] |
− | + | [[Category:Sediment]] | |
− | |||
− | |||
− | |||
− | |||
− | [[Category:Sediment | ||
− |
Latest revision as of 20:46, 15 August 2024
Definition of Marine sediment:
Natural unconsolidated granular material with sediment density greater than water.
This is the common definition for Marine sediment, other definitions can be discussed in the article
|
Contents
Origin of coastal and marine sediments
Continental shelves are formed for the major part from deposits of sediment discharged by rivers. These deposits can reach a thickness of more than 1 km. The sediments have different origins:
Clastic sediments [1]
Clastic sediments (clast = fragment) are ultimate weathering products derived from rock. There are many types of rock, which differ by their origin, crystalline structure and mineral composition:
- Igneous rock = solidified magma. Felsic rock (e.g., granite, rhyolite) is rich in quartz, orthoclase feldspar, amphibole, muscovite, biotite; Mafic rock (e.g., gabbro, basalt) is rich in olivine, pyroxene, anorthite feldspar.
- Sedimentary rock = lithified deposits of mineral or organic particles (e.g., breccia, gritstone, conglomerate, diamict, sandstone, greywacke, arkose, flagstone, siltstone, mudstone, marl)
- Metamorphic rock = sedimentary rock transformed under great heat and pressure beneath the earth crust (e.g., gneiss, slate, marble, schist, quartzite),
Most clastic sediments are terrigenous materials eroded from land. Erosion of rocky shores and submarine rock also produces clastic sediments. About 85% of all coastal marine sediments are clastic sediments[1].
The products of terrestrial rock weathering are transported mainly by rivers (present-time fluvial sediment supply is indicated in Fig. 1), but also by wind, ice and waves. The particles and fragments abrade each other when they are transported by the water flow and erode the surfaces over which they pass, thus contributing to the further breakdown of rock fragments. Time and distance are important factors: the longer the journey to the sea, the more chance there is for mineral grains to be rounded and reduced in size by abrasion. Downstream fining rates strongly differ between rivers[2]. During the downstream journey, grains are sorted according to density, size and shape, and chemically less stable minerals are dissolved. The most common solid products of weathering are rock fragments, quartz and clay minerals. Quartz is the only common rock mineral that is both hard (resistant to abrasion) and chemically stable at the Earth's surface. This is the reason why quartz is the predominant mineral in present day beach and river sands and is also common in most ancient sandstones. The rate at which weathering occurs depends on the local climate, with rapid breakdown in tropical areas, favoured by abundant rainfall and high temperatures, and with slow weathering occurring in deserts, where water is absent. In high-latitude zones, alternating frost and thaw and glacier dynamics are important weathering agents[1].
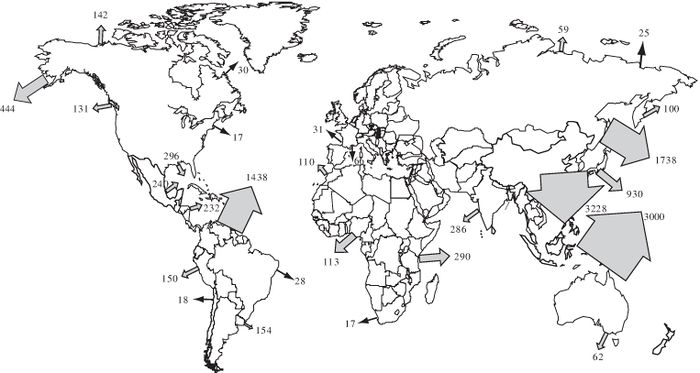
A minor fraction of terrigenous material is deposited from the atmosphere. It consists of dust carried by the wind and ash emitted by volcanoes and contains mainly very fine sand (quartz), but also silt and clay particles with significant amounts of aluminum (Al), iron (Fe), magnesium (Mg) and calcium (Ca ). Supply of atmospheric dust may be a minor factor in most areas today, except from major deserts, e.g., the Sahara, but in the past it has been substantial and has led to the accumulation of extensive loess deposits, e.g., in China. The exceptionally high sediment load of the Yellow river is due to erosion of these loess deposits, which are finally discharged into the Bohai Sea.
In arctic and subarctic areas and where mountains are high enough so as to generate significant quantities of snowfall, glaciers produce substantial amounts of sediment with the meltwater flow through subglacial tunnels.
Clastic sediments can be classified according to grainsize, see Table 1. Grainsizes are indicative of the advancement of the weathering process; small grains derive from weathering of larger grains. Sediment samples generally contain grains of different sizes. Sedimentary deposits are named after the largest present grains (clasts).
particle type | particle name | grainsize d[mm] | grainsize [math]\phi[/math] | lithified |
---|---|---|---|---|
Gravel | Boulders | > 256 | < (-8) | Conglomerate, breccia,
gritstone, diamict |
Cobbles | 64-256 | (-8)-(-6) | ||
Pebbles | 4-64 | (-6)-(-2) | ||
Granules | 2-4 | (-2)-(-1) | ||
Sand | very coarse | 1-2 | (-1)-0 | Sandstone,arkose,
greywacke, flags |
coarse | 0.5-1 | 0-1 | ||
medium | 0.25-0.5 | 1-2 | ||
fine | 0.125-0.25 | 2-3 | ||
very fine | 0.0625-0.125 | 3-4 | ||
Silt | 0.002-0.0625 | 4-9 | Siltstone, mudstone, marl | |
Clay | < 0.002 | > 9 |
Biogenic sediments [4]
Most biogenic sediments are erosion products of unconsolidated or consolidated marine detrital deposits or erosion products of framework-building organisms (e.g. coral reefs). These sediments, called "bioclastic sediments", are composed of fragments of organic skeletal materials . They mainly consist of calcium carbonate (CaCO3) in the form of calcite crystals or aragonite crystals. Other biogenic sediments, such as dead plant remains (peat), have a high organic component and are termed organic-rich biological sediments. Biogenic sediments may constitute a substantial fraction of the bed material in coastal environments where the supply of terrigenous material is small (no nearby river deltas). Many beaches in the tropics and subtropics consist almost entirely of carbonate sands which are derived from adjacent reefs composed of corals, skeletal material, shell fragments or precipitated calcium carbonate. Fine bioclastic sediments may also accumulate in sheltered coastal environments in temperate climate zones. An example is the inner part of the mega-tidal Baie du Mont Saint Michel (almost no fluvial sediment input), where tidal flats are composed of so-called tangue – low-cohesive fine sediment with a high biogenic content [5].
Bioclastic sediments deposited in coastal waters can be lithified into limestone by so-called diagenesis. Thick deposits are cemented under pressure through dissolution of carbonate minerals and subsequent redeposition in the pore spaces. Limestone formation is a slow process that takes place on geological timescales. This contrasts with the decadal timescale of beachrock formation. Beachrock is formed in carbonate-rich nearshore (intertidal) waters, especially in the tropics or subtropics. Limestone fragments, skeletal fragments, quartz sand and other materials are cemented by carbonate precipitation, promoted by geochemical and microbial processes[6].
Chemical sediments
Chemical sediments form by precipitation of minerals out of solution as the water becomes saturated, which is often due to evaporation. The most common chemical sediments formed in this way are calcite (CaCO3), gypsum (CaSO4.2H2O) and halite (NaCl). They are a common type of sediment of sabkhas, back-barrier coastal plains which are ubiquitous in arid climates.
Mineral composition of clastic sediments
Figure 2 shows the average composition by weight of chemical elements in the lithosphere. These elements combine to form minerals. The chief minerals in the lithosphere (earth crust and uppermost mantle) are [7]:
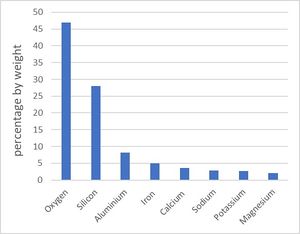
mineral | specific density |
---|---|
Quartz | 2.65 |
Aragonite | 2.95 |
Calcite | 2.71 |
Orthoclase | 2.55-2.63 |
Albite | 2.6-2.65 |
Anorthite | 2.72-2.75 |
Mica | 2.8-3.1 |
Illite | 2.6-2.9 |
Montmorillonite | 1.7-2 |
Vermiculite | 2.4-2.7 |
- Feldspars: Aluminum silicates. The most common forms are: orthoclase KAlSi3O8, albite NaAlSi3O8 and anorthite CaAl2Si2O8. These minerals are fairly hard, often with pink, white, or grey in color. About 40% of the lithosphere consists of feldspars.
- Quartz: silicon dioxide crystal (SiO2);
- Mica: complex silicate minerals comprising elements K or Na and metal cations such as Al, Mg and Fe, that form crystals with a sheet-like arrangement of atoms;
- Clay: hydrous silicates that contain metal cations, generally aluminum. Most common clay minerals are kaolinite (Al2Si2O5(OH)4), illite, smectite (incl. montmorillonite), vermiculite and bentonite. Their basic building blocks are sheets of silica (Si) tetrahedra and oxygen (O) and hydroxyl (OH) octahedra. Micas and clay minerals result originally from chemical weathering of igneous rocks and feldspars. For example, weathering of gabbro or basalt releases smectite, while weathering of granite or rhyolite releases illite[8]. Clay minerals are present in many sedimentary rocks, e.g., sandstone, greywacke, shale, mudstone, siltstone, and slate.
The specific densities (density relative to water [math]s=\rho_{mineral}/\rho_{water}[/math]) of these minerals is given in Table 2.
Physical and chemical properties of sediment
In this section several physical and chemical properties of coastal and marine sediments are shortly introduced. More detailed treatments can be found in other Coastal Wiki articles indicated in the text.
Fall velocity

The seabed of oceans and coastal waters are mainly formed by settling of sediment particles out of suspension. Which particles settle where is mainly determined by the particle fall velocity, concentration and turbulence. Under energetic conditions only coarse grades may settle. The fall velocity of sediment particles depends on size and density. Because the density of different types of sediments is similar (Table 2), the grain size is determinant. The larger the grain size, the higher the fall velocity. The fall velocity as a function of the grainsize is displayed in Fig. 3. Indicated is the fall velocity of round quartz grains in still water at low concentration. In reality, sediment particles do not have a round shape, which slightly affects the fall velocity. The actual fall velocity in flowing water is very different, due to up and down motions caused by turbulent fluid motions ('turbulent eddies'). The concentration of sediment particles also plays a role. At very high concentration, the fall velocity decreases, because particles increasingly interfere with each other, a phenomenon that is called hindered settling[9].
Flocculation
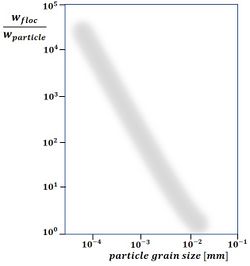
If the fall velocity for very small particles, such as clay minerals, is derived from settling quartz spheres (Fig. 3), it seems so low that they will almost never reach the bottom from suspension. The ubiquitous mud beds in coastal waters point to a different settling mechanism. This mechanism consists of flocculation. Clay minerals have a large surface area relative to their weight, and electrical surface charges. This ensures that they easily bind to one another and to other substances in the water. Clays are therefore classified as cohesive sediments. A substance that serves as a powerful binder is so-called EPS, extracellular polymeric substances [11]. These large organic molecules (polysaccharides, proteins, nucleic acids and lipids) are exuded by living organisms and therefore omnipresent in coastal waters. Flocs therefore grow much faster in natural seawater than in salinized distilled water[12]. In addition to EPS, bacterial colonization also plays a role in flocculation [13]. Flocculation is further influenced by factors such as salinity and pH of the water. When flocs grow, they not only capture smaller flocs that settle more slowly, but also other suspended sediments, such as clay, silt and fine sand. Frequent encounters between sediment particles are important for floc growth. Flocculation is thus enhanced with a high concentration of suspended material and with a certain (low) degree of turbulence (often expressed as the turbulent shear rate [math]G[/math], see below), dependent on the suspended sediment concentration[14]. However, when turbulence is strong (the general case in estuaries), large flocs are broken up.
Flocs settle much faster than the individual constituent particles - a factor of a thousand or more, see Fig. 4. The largest flocs are agglomerates of microflocs. These so-called macroflocs (typical size > 160 [math]\mu[/math]m) have the highest fall velocity, but they are less stable than the smaller microflocs (typical size < 160 [math]\mu[/math]m). The most important factors that determine the fall velocity are the grainsize of the constituent particles, the grainsize of the constituent microflocs, the near-bed shear stress, the suspension concentration and the turbulent shear rate. Further details on flocculation can be found in the article Flocculation cohesive sediments.
Sediment transport
The processes that underlie sediment transport are strongly related to turbulent flow structures in a thin boundary layer near the bed and above. Because these processes are very complicated and not even fully understood, empirical formulas are used in practice for the description of sediment transport. These formulas are described in the articles Sand transport and Sediment transport formulas for the coastal environment. These articles mainly deal with non-cohesive sediments. Transport of cohesive sediments is dealt with in the articles Dynamics of mud transport and Sediment deposition and erosion processes. For measurement of sediment transport see: Measuring instruments for sediment transport, Laboratory and in situ analysis of samples.
Sediment deposits in coastal waters
A more detailed introduction to sediment deposition and erosion is given in the article Sediment deposition and erosion processes.
Deposition of sediment on the seabed takes place when conditions are suitable. These conditions are different for each type of sediment.
When a sediment-laden water mass reaches an area of lower flow strength and wave activity, part of the carried material is deposited. Sediment particles with the greatest fall velocity settle first and particles entrained as bedload (rolling and jumping along the bottom) come to rest. When the current strength and wave activity further decrease, the fine suspended material also settles. In tidal environments, most sedimentation takes place in the period around slack water (flow reversal). Part of the deposited material will afterwards be resuspended by the recovering tidal flow, but another part will remain. This can lead to temporary or permanent deposition. Deposits are temporary if they are insufficiently consolidated and re-eroded during conditions of strong currents (e.g., spring tide) or strong wave action (storm). Permanent deposits have a layered character; each layer represents a deposition period. Successive layers may contain different types of sediment if they have been deposited under different conditions. The layered sediment deposits in estuarine channels sometimes exhibit thin intermediate mud drapes deposited in short periods around high-water or low-water slack tide[15].
Anell (2024[16]) formulated the following empirical rules for inclined sedimentary deposits:
- Deposition occurs as the capacity to transport sediment declines
- Bedform height is generally ruled by accommodation (space available for deposition) and/or energy/agitation
- Smaller bedforms can advance faster to merge with larger bedforms
- Systems can build up to the angle of repose
- The angle of repose is often associated with the formation of linear slopes (or linear segments)
- Coarser grain size is associated with steeper slopes
- Higher mud content is associated with lower slope angles
- Increased pore pressure, associated with high sediment input, can generate failure at low slope angles, where muddy sediment fails at lower angles than sandy sediment
- Sandy slopes tend to be more curved than muddy slopes
- Curvature is an indication of contrast/change along the profile (lithology, grain size, deposition - or lack thereof - or type of depositional process)
- In larger bedforms, steepening with increasing depth is likely the effect of reduced 'relative' sedimentation with increasing accommodation
Graded sediment

Sediment deposits in coastal areas are not always layered; mixed deposits occur frequently. These deposits are referred to as graded sediment. Layered sediment deposits can be mixed when the seabed is strongly perturbated. Mixing also takes place through bioturbation: soil animals (mainly worms and molluscs) bring material from deeper layers to the surface through ingestion and excretion [18][19]. Deposition of mixed sediment also takes place by excretion of filter feeders in the form of fecal pellets. Terminology for mixed deposits is indicated in Fig. 5 for different mixtures of gravel, sand and mud. Mud is itself a mixture of particles with a grain size of less than 0.063 mm, consisting of very fine sand, silt, clay and organic matter.
See also: Biogeomorphology of coastal systems, Sandy shore habitat, Meiofauna of Sandy Beaches.
Vertical segregation, bed armoring
A graded sediment bed stirred by an oscillatory current can undergo inverse grading by a process called 'kinematic vertical sorting', see Seabed armoring. Coarse sediments are lifted gradually to the seabed surface, leading to the formation of a coarse armor top layer that covers a sublayer of finer sediment. These finer sediments are therefore better protected from erosion.
Horizontal segregation
Spontaneous horizontal segregation of graded sediment can occur under certain conditions. This segregation generates contiguous patches of different types of sediment. The underlying feedback mechanism links sedimentation of fine material on a smooth muddy seabed to a locally reduced degree of turbulence [20]. As a result, fine material will mainly deposit in places where fine material already dominates on the seabed, so that these muddy patches increase in size and in mud content. This continues until no fine material is available any more from neighboring patches of coarser deposits from which the fines have been winnowed.
Bedforms
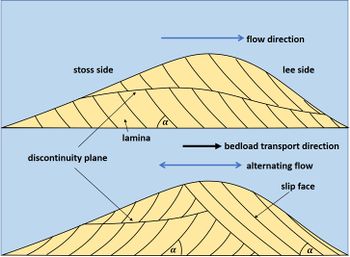
Sediment layers are generally horizontal, but can also have a wavy character. These undulations are caused by the fact that the interaction between flow and sediment bed does not behave linearly, meaning that small disturbances of the flat sediment bed can start growing exponentially. This leads to the emergence of a large range of bedforms, as explained in the article Stability models. The smallest bedforms, ripples, arise in places where bed sediments are sandy and where currents and wave activity are not very strong but sufficient to set sediment in motion. This is explained in the articles Wave ripples and Wave ripple formation, for situations where sediment movement is mainly determined by waves. When wave activity increases, while the background current remains small, ripples can transform into patchy seabed structures called hummocks [22]. Bed ripples exert friction on tidal currents and have a great influence on the flow velocity. More details can be found in the articles Bedforms and roughness and Bed roughness and friction factors in estuaries. In addition to ripples, much larger bedforms can also arise, for example: megaripples (wave length of several meters), transverse and longshore bars (wave length of the order of one hundred meters, see Rhythmic shoreline features), dunes (also called sandwaves, wavelength of tens to hundreds of meters), cheniers (sand ridges on muddy shorelines, wavelength of the order of one hundred meters[23]) and shoreface-connected ridges and tidal ridges (wavelength of several kilometers, see Sand ridges in shelf seas). Different bedforms scales mentioned may be found superimposed on each other. In the underlying sedimentary layers, bed ripples are not always preserved, but the larger bedforms can generally be observed quite well.
The typical layer structure of the seabed that occurs under the stoss slope of migrating bedforms, such as (mega)ripples or dunes, is called cross-stratification. The layer structure consists of parallel laminae formed by sediment avalanching at the migrating bedform crest (more precisely, at the brinkpoint, sligtly downstream of the crest). The laminae are strongly dipping in the direction of bedform migration, according to the angle repose, see Fig. 6.
The bedforms described above do not occur with deposits of fine cohesive material (mud). Dewatering of freshly deposited mud is a slow process and mud layers therefore remain fluid for a long time as so-called fluid mud, see for example the article Dynamics of mud transport. Once consolidated, the erosion resistance is high, so that no bed ripples can form. Mud layers therefore have a smooth surface and exert little friction on the flow.
Large-scale sediment sorting
Sediment sorting, the spatial segregation of different types of sediment deposits, is a characteristic of mobile sediment beds. As indicated earlier, sediment deposition requires suitable hydrodynamic conditions, which depend on the type of sediment. Fine sediments cannot settle in high-energy environments (strong waves, strong currents). Temporary settling is possible when currents are weak (neap tide, slack tide) and in the absence of strong wave action. However, in situations where low-energy and high-energy conditions alternate, these temporary deposits will disappear. Although currents generally carry a mixture of different types of sediment, remaining deposits will only comprise grainsizes that cannot be resuspended under the most energetic conditions among the alternating conditions that occur at a specific location. Grain size analysis of sediment deposits therefore can provide an indication of the maximum hydrodynamic shear stresses that occur at a particular location[24][25]. A qualitative overview of the types of sediment deposits that form the seabed top layer under different hydrodynamic conditions is indicated in Table 3 for sandy coastal environments, together with the associated sediment transport modes and bedforms. These bedforms are themselves cause of sediment sorting. In low-energetic environments (weak currents, waves) the coarsest sediments accumulate in the troughs (swales) by preferential downward motion along the slip face of the bedforms[22]. In the case of stronger waves and currents the coarsest sediments are found at the bedform crest where fine sediments are most easily brought in suspension [26].
Hydrodynamic conditions | Sediment | Bedform | Sediment transport mode |
---|---|---|---|
Strong currents [math]\small U_c \gt 1 m/s[/math] | Medium-coarse sand | 3D dunes | Suspended load |
Medium currents [math]\small 0.5\lt U_c\lt 1 m/s[/math] | Fine-medium sand, mud drapes | Ripples, 2D dunes | Bedload, suspended load |
Weak currents [math]\small U_c\lt 0.5 m/s[/math] | Mud, fine sand | Flat bed (mud), ripples (fine sand) | Suspended load (mud), bedload (fine sand) |
Strong waves [math]\small U_w\gt 1 m/s[/math] | Fine-coarse sand | Flat bed | Suspended load, sheet flow |
Medium waves [math]\small 0.3\lt U_w\lt 1 m/s[/math] | Fine-medium sand, mud drapes | Wave ripples | Bedload, suspended load |
Weak waves, currents [math]\small U_w\lt 0.3 m/s, U_c\lt 0.5 m/s[/math] | Fine sand, mud layers | Wave ripples (fine sand), flat bed (mud) | Bedload, suspended load |
Strong currents, strong waves [math]\small U_c, U_w\gt 1 m/s[/math] | medium-coarse sand | Flat bed | Suspended load, sheet flow |
Seabed erosion
When exposed to turbulent shear stresses due to currents or waves, sediment particles are dislodged from the seabed and lifted one by one or in lumps. For this, the shear stress must exceed a certain critical value, [math]\tau_{cr}[/math], which depends on many factors, in particular the grainsize (see Fig. 7). The white gap in Fig. 7 shows that seabed erosion is influenced by other factors than shear stress and grain size. These factors are[28]:
- Specific density. The sediment specific density is not considered in this figure, because it is rather similar for most mineral sediments. This does not apply to sediments of biotic origin, in which case besides density, biochemical bonds also strongly affect erodibility.
- Angularity of sediment particles. Rounded grains are less susceptible to uptake than angular grains[29].
- Sediment sorting. When sediment deposits are a mixture of coarse and fine sediment, the surface layer will mainly consist of coarse particles that protect the underlying fine sediment from erosion (so-called bed armoring effect). However, the coarse grains will be more exposed and are therefore more easily expelled from the sediment bed.
- Peak turbulent intensity. The shear stress represents the average turbulent intensity. However, seabed erosion is sensitive to incidental peaks of high turbulent intensity (associated with turbulent bursting events). Some seabed erosion therefore can occur already at quite low values of the shear stress[30].
- Consolidation. Fine sediment can be easily resuspended when it has just been deposited. Freshly deposited fine sediment has a large pore volume filled with seawater that can be set in motion under the influence of horizontal pressure gradients of the surface water. Over time, the seawater is forced out of the pores by the weight of the consolidating deposit. The bulk density of the deposit increases while the permeability decreases. This causes a sharp increase of the critical shear stress for erosion[31].
- Clay content of the deposit. The molecular structure of clay particles enables the formation of electrochemical bonds that significantly increase the resistance of the deposit against erosion. This effect already occurs at a clay content of just 10%. A deposit with a sufficiently high clay content retains the water for a long time and consolidates slowly. Once consolidated, the deposit forms a hard layer that can withstand very high shear stresses. See Sediment deposition and erosion processes for further details.
- Biota. Living organisms can strongly alter the erosion strength of seabed deposits. Various large organic molecules, called EPS (extracellular polymeric substances), secreted by micro-organisms, bind sediment particles together and form biofilms at the sediment surface, especially in intertidal areas. Erosion resistance of sediment deposits is also favored by the formation of microbial mats, often called algal mats. Erosion resistance is diminished by other organisms, bivalves in particular, that disturb the sediment bed by so-called bioturbation and graze on microalgae. More details can be found in Biogeomorphology of coastal systems.
The amount of sediment [math]E[/math] [kg s-1m-2] that is brought in suspension when the shear stress [math]\tau[/math] exceeds the critical stress [math]\tau_{cr}[/math] for initiation of erosion, primarily depends on the excess shear stress [math]\tau - \tau_{cr}[/math]. However, it also depends on many other factors. For fine non-cohesive sand, the relation can be represented by the empirical formula[32]
[math]E = M \, (\Large\frac{\tau}{\tau_{cr}}\normalsize -1)^n ,[/math]
with [math]n \sim [/math] 1 and [math]M \sim [/math] 0.05-0.1 kg m-2s-1. For fine cohesive sediment, [math]M[/math] and [math]n[/math] strongly depend on the degree of consolidation and must be determined experimentally. The values found for [math]n[/math] are generally much larger than 1 and the values of [math]M[/math] much smaller than 0.1 kg m-2s-1, see Sediment deposition and erosion processes for further details. In practice, the coefficients [math]M[/math] and [math]n[/math] change with time because the consolidation degree of the seabed changes with depth.
Sediment contamination and bioavailability
As indicated earlier, fine sediments - clay particles in particular - can easily bind with other substances in the water. This binding is called sorption: adsorption (=surface binding) or absorption (=uptake). This property allows fine sediments to filter out dissolved contaminants from the water. The water becomes less polluted, but the contamination of bed sediments, on the other hand, increases. The partition of pollutants between the dissolved phase and the sediment-bound phase is represented by the parameter [math] K_d [/math]. This partition, i.e. the value of [math] K_d[/math], depends on the type of pollutant and the type of sediment.
Laboratory tests show that for inorganic contaminants, for example heavy metals such as lead (cation Pb2 +), cadmium (Cd2 +) and copper (Cu2 +), the partition depends on the sediment grainsize [33]. The smaller the grainsize (i.e. greater surface/volume), the stronger is the sorption (large [math] K_d [/math]), almost independently of the concentration of dissolved heavy metals. Biogeochemical processes determine the degree to which trace metals (especially cadmium and copper) are bound to sediment, depending on several factors, such as salinity and oxygen content. In an estuary, the ratio between the bound and labile (bioavailable) fraction of trace metals is variable in space and time. For example, measurements in the Scheldt estuary show that the labile fraction is greatest in the salt-fresh transition zone[34].
For organic pollutants such as PCB s (polychlorinated biphenyls) and PAH s (polyciclic aromatic hydrocarbons), sorption to sediment is primarily determined by the organic carbon content in the sediment, rather than by the grain size[35]. This is a well-known feature in water sanitation technology, where active carbon is used to filter contaminants from waste water.
Contaminants are much less toxic to marine organisms when they are bound to sediment than when they are dissolved in water [36]. The bioavailability decreases further as the sediment bed gets older. Perturbation of the sediment bed by dredging or by bioturbation, however, plays a role. When sediment is worked up from a deeper anoxic layer to a higher oxic layer, attached metals are released by desorption. Burial of sediments to deeper soil layers, on the other hand, reduces bioavailability. The bioavailability of contaminants in soils can be reduced by adding active carbon[36].
Spatial distribution of sediments
The type of sediment occurring on the seafloor varies greatly from place to place. The presence of nearby sediment sources plays an important role, but hydrodynamic conditions strongly influence which sediments are deposited where. Because certain types of sediment are only deposited under specific conditions, sorting occurs of the different types of sediment that are supplied. In the following, a short description is given of resulting sediment deposits in different marine environments.
Oceans

The deep oceans have four main sediment sources [4] (Fig. 8):
- Clastic fluvial sediments. Large quantities of fine sediments (silt, clay and fine sand) are deposited by the world's major rivers at the edges of the continental shelfs. These deposits destabilize the continental shelf slope, causing the sediment to slide down to the ocean floor by slumping or through gravity currents. These deposits are confined along the shelf break and are not further spread over the ocean floor.
- Atmospheric dust. A large part of the fine sediments entrained by wind from arid land areas and ash from volcanic eruption plumes is deposited in the oceans (e.g., red clay deposits, manganese nodules). This contribution to ocean sedimentation is relatively small nowadays.
- Carbonaceous ooze. The remains of calcareous algae, mainly coccoliths and foraminifera, are a major component of the seafloor sediment in large parts of the ocean. Below depths of 3 to 4 km, however, they do not occur, because the scales dissolve here as a result of too high a pressure and too high a concentration of carbon dioxide.
- Siliceous ooze. The remains of silicate plankton, mainly diatoms and radiolaria, dominate in other parts of the ocean - especially in the central part of the Pacific and in the Antarctic Ocean.
Beach and foreshore

Sediments on the foreshore and subaerial beach are derived from nearby rivers or from ancient offshore river deposits. The foreshore is a highly energetic environment due to the activity of waves, which prevent settling of fine sediments. Sand and gravel are the dominant seabed sediments, depending on the supply of these sediments. The coarsest sediments are found in the breaker zone, especially in the higher part where the waves collapse on the beach. The finer fractions are found in the offshore zone and also on the backshore and the dunes, which are fed from the dry beach by aeolian transport. The type of sediment on the shoreface largely determines the equilibrium shoreface slope for a given wave climate. The relationship between sediment grain size, wave climate and beach slope can be characterized by the Dean parameter
[math]\Omega = H / (w T) , [/math]
where [math]H[/math] is the significant offshore wave height (before breaking), [math]w[/math] the sediment fall velocity and [math]T[/math] the peak wave period. This relationship is schematically shown in Fig. 9, which holds for sandy or gravelly coasts.

Figure 9 suggests a positive correlation between sediment grainsize and beach slope (the beach slope is defined here as the average slope of the beach face, the zone between the low-water line and the beach berm). This is substantiated by a study of Bujan et al. (2019)[38], who compiled data on grainsize and associated beach slope ([math]\beta[/math]) from a large number of field sites. The result is shown in Fig. 10, where the violet area represents more than 95% of the data points. The dark blue line is a fit to the observed values represented by the empirical relation [math]\tan \beta = -0.154(D_{50}-0.125)^{-0.145} + 0.268[/math]. The large bandwidth around this line reflects the different characteristics of the field sites (wave climate, tides, beach profile, sediment sorting, ...) and the different data collection and analysis methods used in the underlying studies. Grainsize and beach slope are positively correlated. For large grainsizes, wave up- and downrush is reduced by infiltration into the the sediment bed. This is a plausible explanation for the weak dependence of beach slope on grainsize for coarse sediment, compared to the strong dependence for fine-medium sediment[38].
For a more detailed discussion of the shoreface equilibrium profile the reader is referred to the article Shoreface profile, which deals with sandy coasts.
Coasts with abundant fine sediment supply are not well represented by the Dean parameter and the grainsize-beach slope relationship of Fig. 10. Large deposits of fine sediments can occur on the foreshore and upper shoreface of such coasts. These deposits absorb most of the energy of incident waves into a fluid mud layer which is maintained by absorption of wave energy. A more detailed description is given in the article Coastal mud belt.
Estuaries
The sediment distribution in estuaries is particularly complex. Sediment deposits depend on the supply of river sediment, the supply of sediment from the sea and on local flow and wave conditions, in relation with a generally intricate topography. In addition, deposits are influenced by strong fluctuations in river discharge, tides and wave conditions at various timescales. Nevertheless, the sediment distribution in estuaries generally displays a characteristic pattern that is shown in Fig. 11 and discussed below.

Gravel transported by rivers is mostly deposited upstream and does not reach coastal plain estuaries. This is the case when catchment areas are situated far from the sea; long rivers discharge mainly sandy and muddy sediments into broad coastal plain estuaries. In the case of mountainous coasts, estuaries are generally small drowned river valleys (rias); they may receive substantial amounts of gravely sediments, which are further discharged to the coastal zone by strong river floods. Most coastal plain estuaries also receive sand and mud imported from the sea by tidal flood currents. The estuarine morphology is in morphodynamic equilibrium if, averaged over a long period, the inflow of sand and mud is balanced by export to the sea by outflowing ebb currents[40], see Morphology of estuaries. This is not a static equilibrium, because sand and mud are moving across the estuary all the time, including temporal deposition and erosion.
Morphodynamic equilibrium does not imply that the concentration of suspended sediment in an estuary is uniform. Concentration maxima occur in convergence zones where temporal settling of sediments takes place (or ongoing settling in the case of non-equilibrium). These convergence zones depend not only on flow strength and flow pattern, but also on transport, settling and erosion characteristics of sediment particles. The areas of highest suspended concentration and deposition thus differ for sand and mud [41].
Convergence of sand transport takes place on the outer delta, where the ebb flow expands and decelerates. Within the estuary, convergence zones of sand transport are marked by sand banks and sand bars which have grown at the junctions of ebb and flood flow channels and at the inner bends of flow meanders.
Convergence of mud transport (silt and clay) occurs in the transition zone between flood dominant near-bed flow (due to tidal asymmetry and estuarine density-driven circulation) and ebb dominant flow (river flow enhanced by ebb tidal currents). This transition zone has the highest mud concentrations, both in the water column (turbidity maximum) and in the channel bed (for more details, see the articles Estuarine turbidity maximum, Dynamics of mud transport, Sediment deposition and erosion processes). Besides, mud concentrations are high in sheltered areas where fine sediments can settle without immediately being resuspended, such as salt marshes and mangroves. In these areas, waves and currents are strongly reduced by vegetation, which enhances sediment deposition. See also: Dynamics, threats and management of salt marshes and Mangroves.
Related articles
- Sediment deposition and erosion processes
- Dynamics of mud transport
- Shoreface profile
- Estuarine turbidity maximum
- Coastal mud belt
- Wave-induced soil liquefaction
References
- ↑ 1.0 1.1 1.2 1.3 1.4 Huggett, R.J. 2007. Fundamentals of geomorphology. Routledge, Taylor & Francis
- ↑ Reyolds, T. 2024. Grain size from source to sink – modern and ancient fining rates. Earth-Science Reviews 250, 104699
- ↑ Milliman, J. D. and Meade, R. H. 1983. World-wide delivery of sediment to the oceans. Journal of Geology 91, 1–21
- ↑ 4.0 4.1 Taylor, K.G. 2008. Sediments and sedimentation. In: An Introduction to Physical Geography and the Environment (J. Holden, editor), Pearson Education Limited
- ↑ Desguée, R., Robin, N., Gluard, L., Monfort, O., Anthony, E.J. and Levoy, F., 2011. Contribution of hydrodynamic conditions during shallow water stages to the sediment balance on a tidal flat: Mont-Saint-Michel bay, Normandy, France. Estuarine, Coastal and Shelf Science, 94, 343-354
- ↑ Vousdoukas, M.I., Velegrakis, A.F. and Plomaritis, T.A. 2007. Beachrock occurrence, characteristics, formation mechanisms and impacts. Earth-Science Reviews 85: 23–46
- ↑ Anderson, R.S. and Anderson, S.P. 2010. Geomorphology: The Mechanics and Chemistry of Landscapes. Cambridge University Press. p. 187.
- ↑ Zhao, T., Xu, S. and Hao, F. 2023. Differential adsorption of clay minerals: Implications for organic matter enrichment. Earth-Science Reviews 246, 104598
- ↑ Winterwerp, J.C. 2002. On the flocculation and settling velocity of estuarine mud. Continental Shelf Research 22: 1339–1360
- ↑ Migniot, C. 1968. A study of the physical properties of various forms of very fine sediments and their behaviour under hydrodynamic action. La Houille Blanche 7, 591–620
- ↑ Grabowski, R.C., Droppo, I.G. and Wharton, G. 2011. Erodibility of cohesive sediment: the importance of sediment properties. Earth Science Reviews 105 (3-4): 101-12
- ↑ Skinnebach, K.H., Fruergaard, M. and Andersen, T.J. 2019. Biological effects on flocculation of fine-grained suspended sediment in natural seawater. Estuarine, Coastal and Shelf Science 228, 106395
- ↑ Linley, E.A.S. and Field, J.G. 1982. The nature and significance of bacterial aggregation in a nearshore upwelling ecosystem. Estuarine, Coastal and Shelf Science 14: 1-11
- ↑ Mietta, F., Chassagne, C., Manning, A.J. and Winterwerp, J.C. 2009. Influence of shear rate, organic matter content, pH and salinity on mud flocculation. Ocean Dynamics 59: 751–763
- ↑ 15.0 15.1 Martinius, A.W. and van den Berg, J.H. 2011. Atlas of sedimentary structures in estuarine and tidally influenced river deposits of the Rhine-Meuse-Scheldt system. EAGE Publ. ISBN 978-90-73834-11-8
- ↑ Anell, I. 2024. The quintessential s-shape in sedimentology: A review on the formation and controls of clinoform shape. Earth-Science Reviews 254, 104821
- ↑ Blair, T.C. and McPherson, J.G. 1999. Grain-size and textural classification of coarse sedimentary particles. Journal of Sedimentary Research 69: 6–19
- ↑ Baumfalk, Y.A. 1979. Heterogeneous grain size distribution in tidal flat sediment caused by bioturbation activity of Arenicola marina (polychaeta). Netherlands Journal of Sea Research 13: 428-440
- ↑ Gallagher, E.D. 2008. Bioturbation. Biol. Ocean. Processes, EEOS 630
- ↑ Murray, A.B. and Thieler, E.R. 2004. A new hypothesis and exploratory model for the formation of large-scale inner-shelf sediment sorting and ‘rippled scour depressions’. Continental Shelf Res. 24: 295-315
- ↑ Reineck, H.-E. and Singh, I.B. 1973. Depositional sedimentary environments. Springer, Berlin, 439 pp.
- ↑ 22.0 22.1 Van den Berg, J.H. and Nio, S.D. 2010. Sedimentary structures and their relation to bedforms and flow conditions. EAGE Publications
- ↑ Augustinus, P.G.E.F. 1989. Cheniers and chenier plains: a general introduction. Marine Geology 90: 219-229
- ↑ Ward, S.L., Neill, S.P., Van Landeghem, K.J.J. and Scourse, J.D. 2015. Classifying seabed sediment type using simulated tidal-induced bed shear stress. Marine Geology 367: 94–104
- ↑ Escobara, C.A., Mayerle, R. and Restrepo, D. 2019. Estimation of sediment grain sizes in a mesotidal area, Dithmarschen Bight, German North Sea. Marine Geology 417, 106006
- ↑ Van Oyen, T., Blondeaux, P. and Van den Eynde, D. 2013. Sediment sorting along tidal sand waves: A comparison between field observations and theoretical predictions. Continental Shelf Research 63: 23–33
- ↑ Terwindt, J.H.J. 1981. Origin and sequences of sedimentary structures in inshore mesotidal deposits of the North Sea. Spec. Publs. Int. Ass. Sediment. 5: 4-26
- ↑ Yang, Y., Gao, S., Wang, Y.P., Jia, J., Xiong, J. and Zhou, L. 2019. Revisiting the problem of sediment motion threshold. Continental Shelf Research 187, 103960
- ↑ Paphitis, D. 2001. Sediment movement under unidirectional flows: an assessment of empirical threshold curves. Coastal Engineering. 43: 227-245
- ↑ Mohtar, W.H.M.W., Lee, J.W., Azha, N.I.M. and Cheng, N-S. 2020. Incipient sediment motion based on turbulent fluctuations. International Journal of Sediment Research 35: 125-133
- ↑ Mohr, H., Draper, S., White, D.J. and Cheng, L. 2021. The effect of permeability on the erosion threshold of fine-grained sediments. Coastal Engineering 163, 103813
- ↑ Mehta, A. J. and Partheniades, E. 1982. Resuspension of deposited cohesive sediment beds. Procs. 18th International Conference on Coastal Engineering, Cape Town, South Africa
- ↑ Schorer, M. 1997. Pollutant and organic matter content in particle size fractions. Freshwater contamination, IAHS publ. 243.
- ↑ Gaulier, C., Zhou, C., Gao, Y., Guo, W., Reichstädter, M., Ma, T. Baeyens, W. and Billon, G. 2021. Investigation on trace metal speciation and distribution in the Scheldt estuary. Science of the Total Environment 757, 143827
- ↑ Karickhoff, S.W., Brown, D.S. and Scott, T.A. 1979. Sorption of hydrophobic pollutants on natural sediments. Water Research 13: 241-248
- ↑ 36.0 36.1 National Research Council. 2003. Bioavailability of Contaminants in Soils and Sediments: Processes, Tools, and Applications. Washington, DC: The National Academies Press. doi: 10.17226/10523
- ↑ Flemming, B.W. and Fricke, A.H. 1983. Beach and nearshore habitats as a function of internal geometry, primary sedimentary structures and grain size. In: McLachlan, A., Ersamus, T. (Eds.), Sandy Beaches as Ecosystems. Dr. W. Junk Publishers, The Hague, pp. 115–132
- ↑ 38.0 38.1 38.2 Bujan, N., Cox, R. and Masselink, G. 2019. From fine sand to boulders: Examining the relationship between beach-face slope and sediment size. Marine Geology 417, 106012
- ↑ Dalrymple, R.W., Zaitlin, B.A., Boyd, R., 1992. Estuarine facies models: conceptual basis and stratigraphic implications. Journal of Sedimentary Petrology 62, 1130–1146
- ↑ Dronkers, J. 2017. Convergence of estuarine channels. Cont. Shelf Res. 144: 120-133
- ↑ Dronkers, J. 2017. Dynamics of coastal systems. World Scientific Publ. Co., 753 pp.
Please note that others may also have edited the contents of this article.
|