Difference between revisions of "Submarine groundwater discharge"
Dronkers J (talk | contribs) |
Dronkers J (talk | contribs) |
||
(3 intermediate revisions by the same user not shown) | |||
Line 1: | Line 1: | ||
− | '''Submarine groundwater discharge''' (SGD) can have a significant influence on the coastal environment. This article examines the underlying processes and the importance for coastal zone management. In order to assess the magnitude of SGD, different investigations based on models and on direct measurements have been carried out. Indirect indicators of SGD are also considered. | + | '''Submarine groundwater discharge''' (SGD) can have a significant influence on the coastal environment. This article examines the underlying processes and the importance of submarine groundwater discharge for coastal zone management. SGD is the discharge of groundwater going directly into the sea, and not via surface streams. Groundwater is synonymous with soil pore water. In order to assess the magnitude of SGD, different investigations based on models and on direct measurements have been carried out. Indirect indicators of SGD are also considered. |
==Process description and estimates== | ==Process description and estimates== | ||
+ | Submarine groundwater discharge (SGD) is defined as the discharging flow out of the aquifer across the sea floor <ref name="Burnett">Burnett, W.C., et al. (2006) Quantifying submarine groundwater discharge in the coastal zone via multiple methods. ''Sciences of the Total Environment'', '''367''' 498-543.</ref>. It is a process complementary to saltwater intrusion, which is defined as the invasion of seawater at the lower part of the aquifer due to the density difference between fresh groundwater and seawater, see [[Groundwater management in low-lying coastal zones]]. The processes are shown schematically in Figures 1a and 1b. | ||
− | + | If mixing between fresh groundwater and the seawater wedge is weak, it can be assumed that the interface between the two areas is sharp (Figure 1a). In such cases, the submarine fresh groundwater flows to the sea above the saltwater wedge. During the winter period the interface is located more landward than in summer, assuming that the groundwater levels occurring in fall are relatively low. During summer, the interface moves seaward, assuming a rise of the groundwater level during spring, while, simultaneously with fresh groundwater discharge, seawater outflow takes place from the saltwater wedge. The seawater outflow is generally much greater than the fresh groundwater discharge<ref name="Michael">Michael H.A., Mulligan A.E. and Harvey C.F. (2005) Seasonal oscillations in water exchange between aquifers and the coastal ocean. ''Nature'' '''436''' 1145-1148.</ref>. | |
− | + | [[File:Exchange fluxes for sharp interface.PNG|thumb|frame|caption| Fig 1a. Exchange fluxes in case of sharp interface between groundwater and seawater for high and low groundwater levels]] | |
− | + | In general there is no sharp interface between the freshwater and the saltwater. Due to [[dispersion]] and molecular diffusion a freshwater-seawater mixing zone is built between the two areas. The mixing causes the salt water in the deeper part of the aquifer to become lighter, rise up and discharge back to the sea (Figure 1b). In this case, SGD consists of a mixture of fresh groundwater and recirculated seawater. The recirculation of seawater, in the nearshore part of the aquifer, is further enhanced by mechanisms acting from the marine side such as tidal pumping and wave set-up. The time variation of SGD exhibits components at small time scales (minutes, hours, days) as well as components of large time scale, which are due to the seasonal motion of the mixing zone and large-scale sea level variations. Because aquifer recharge is faster than aquifer discharge, seawater infiltration by waves and tides creates an over-height of the groundwater table (pumping effect). This over-height can exceed, during storm conditions, all other geophysical drivers of fluid flow across the sediment-water interface (Sawyer et al., 2013<ref>Sawyer A. H., Shi F., Kirby J. T. and Michael H. A. (2013). Dynamic response of surface water-groundwater exchange to currents, tides, and waves in a shallow estuary. J. Geophys. Res. C '''118''' 1749–1758</ref>). | |
− | |||
− | [[Image:Exchange fluxes | + | [[Image:Exchange fluxes mixing.jpg|thumb|frame|caption| Fig 1b Exchange fluxes in case of mixing between fresh groundwater and seawater]] |
Concerning the contribution of the fresh groundwater flow and the seawater recirculation to the total SGD, it is estimated that: | Concerning the contribution of the fresh groundwater flow and the seawater recirculation to the total SGD, it is estimated that: | ||
− | * The global total SGD flux, which includes recirculated seawater, could be three to four times larger than the global river flux, based on measured radium isotope concentrations in seawater<ref name=Kwon>Kwon, E. Y., Kim, G,. Primeau, F., Moore, W.S., Cho, H.-M., DeVries, T., Sarmiento, J. L., Charette, M. A. and Cho, Y.-K. (2014) Global estimate of submarine groundwater discharge based on an observationally constrained radium isotope model. Geophys. Res. Lett. '''41''' 8438–8444, doi:10.1002/2014GL061574</ref>. The terrestrially derived SGD was estimated by Burnett et al. (2003<ref name="Burnett.">Burnett, W.C., et al. (2003) Groundwater and pore water inputs to the coastal zone. ''Biogeochemistry'' '''66''' 3-33.</ref>) in the range of 6-10% of the surface waters discharging into the ocean. However, more recent calculations by Luijendijk et al. (2020<ref name=LGM>Luijendijk, E., Gleeson, T. and Moosdorf, N. (2020) Fresh groundwater discharge insignificant for the world’s oceans but important for coastal ecosystems. Nature Commun. '''11''' 1260. https://doi.org/10.1038/s41467-020-15064-8</ref>) show that the submarine groundwater discharge cannot exceed 5.5% of the river input into the world’s oceans, assuming that all groundwater recharge in coastal watersheds were to discharge directly into the oceans. Sawyer et al. (2016<ref>Sawyer, A.H., David, C.H. and Famiglietti, J.S. (2016) Continental patterns of submarine groundwater discharge reveal coastal vulnerabilities. Science '''353''' 705–707</ref>) estimate that the total volumetric rate of fresh SGD from the contiguous United States to the oceans may | + | * The global total SGD flux, which includes recirculated seawater, could be three to four times larger than the global river flux, based on measured radium isotope concentrations in seawater (Kwon et al., 2014<ref name=Kwon>Kwon, E. Y., Kim, G,. Primeau, F., Moore, W.S., Cho, H.-M., DeVries, T., Sarmiento, J. L., Charette, M. A. and Cho, Y.-K. (2014) Global estimate of submarine groundwater discharge based on an observationally constrained radium isotope model. Geophys. Res. Lett. '''41''' 8438–8444, doi:10.1002/2014GL061574</ref>.) The terrestrially derived SGD (fresh water) was estimated by Burnett et al. (2003<ref name="Burnett.">Burnett, W.C., et al. (2003) Groundwater and pore water inputs to the coastal zone. ''Biogeochemistry'' '''66''' 3-33.</ref>) in the range of 6-10% of the surface waters discharging into the ocean. However, more recent calculations by Luijendijk et al. (2020<ref name=LGM>Luijendijk, E., Gleeson, T. and Moosdorf, N. (2020) Fresh groundwater discharge insignificant for the world’s oceans but important for coastal ecosystems. Nature Commun. '''11''' 1260. https://doi.org/10.1038/s41467-020-15064-8</ref>) show that the submarine groundwater discharge of terrestrial origin cannot exceed 5.5% of the river input into the world’s oceans, assuming that all groundwater recharge in coastal watersheds were to discharge directly into the oceans. Sawyer et al. (2016<ref>Sawyer, A.H., David, C.H. and Famiglietti, J.S. (2016) Continental patterns of submarine groundwater discharge reveal coastal vulnerabilities. Science '''353''' 705–707</ref>) estimate that the total volumetric rate of fresh SGD from the contiguous United States to the oceans may amount to 25 ± 7 cubic km/year, or 1-2% of surface runoff. |
− | * Assuming that recirculation is only due to density effects and mixing, recirculated seawater can be up to 70% of the SGD <ref name="Smith">Smith A.J. (2004) Mixed convection and density-dependent seawater circulation in coastal aquifers. ''Water Resource Res.'', '''40''' W08309. doi:10.1029/2003WR002977 | + | * Assuming that recirculation is only due to density effects and mixing, recirculated seawater can be up to 70% of the SGD (Smith, 2004<ref name="Smith">Smith A.J. (2004) Mixed convection and density-dependent seawater circulation in coastal aquifers. ''Water Resource Res.'', '''40''' W08309. doi:10.1029/2003WR002977</ref>; Kaleris, 2006<ref name="Kaleris">Kaleris V. (2006) Submarine groundwater discharge: Effects of hydrogeology and of nearshore surface water bodies. ''J. Hydrology'', '''325''' 96-117.</ref>); |
− | * Assuming that recirculation is also due to wave setup and tide, the recirculated sea water may constitute up to 96% of the SGD <ref name="Li">Li L. | + | * Assuming that recirculation is also due to wave setup and tide, the recirculated sea water may constitute up to 96% of the SGD (Li et al. 1999<ref name="Li">Li L., Barry D. A., Stagnitti F. and Parlange J.-Y. (1999) Submarine groundwater discharge and associated chemical input to a coastal sea. ''Water Resour. Res.'' '''35'''(11) 3253-3259</ref>). However, Luijendijk et al.<ref name=LGM/> estimate that the fresh SGD is even a much smaller fraction, 0.06% (0.0003%–0.2%), of the global total SGD flux, which thus consists almost entirely of recirculated seawater. According to these authors, the contribution of fresh groundwater discharge to the oceans is globally in the order of ~0.6% (0.004%–1.3%) of the total freshwater input and ~2% (0.003%–7.7%) of the solute input for carbon, nitrogen, silica and strontium. |
+ | |||
+ | ==Submarine groundwater pathways== | ||
[[File:Submarine_Groundwater_4.jpg|thumb|left|350px|Fig. 2. Example of “sand boils”, where freshwater is flowing out of the sediment.]] | [[File:Submarine_Groundwater_4.jpg|thumb|left|350px|Fig. 2. Example of “sand boils”, where freshwater is flowing out of the sediment.]] | ||
− | |||
− | |||
− | + | Submarine groundwater flow is usually three dimensional and highly complex due to the patchy nature of burrows, subtle geomorphological gradients, and heterogeneous sediments and vegetation. It is distributed over large areas, but particularly strong, concentrated, submarine groundwater discharges occur in case of karstic aquifers discharging into the sea (karstic submarine springs). Fault lines or fracture patterns and submarine paleochannels, infilled with permeable sediments, are also preferential pathways of SGD (Taniguchi et al., 2019<ref name=T19>Taniguchi M., Dulai H., Burnett K.M., Santos I.R., Sugimoto R., Stieglitz T., Kim G., Moosdorf N. and Burnett W.C. (2019) Submarine Groundwater Discharge: Updates on Its Measurement Techniques, Geophysical Drivers, Magnitudes, and Effects. Front. Environ. Sci. '''7''' 141</ref>). Submarine groundwater discharge can take place not only near the shore but also at great offshore distances. The latter occurs when confined aquifers, underneath the continental shelf, have their outcrop on the sea bottom and at a large distance from the shore. Concentrated outflows at the sea bottom occur in cases where the outcrops of alluvial aquifers are of limited area. Such outflow locations on the sea bottom sometimes form pockmarks in the fine-grained sediments of the sea floor, see Fig. 2. | |
+ | |||
+ | Coastal groundwater discharge hotspots cover 9% (0.02–30)% of the global coastline and are predominantly located in areas with a steep coastal topography due to glacio-isostatic rebound, active tectonics or volcanic activity and areas consisting of permeable unconsolidated sediments, carbonates or volcanic rocks. The distribution of hotspots is consistent with documented sites of high fresh groundwater discharge globally that are predominantly located in North America, Europe, and East Asia (Luijendijk et al., 2019<ref name=LGM/>). A list of SGD hotspots from field studies reported in the literature is presented in Nakajima et al. (2024<ref>Nakajima, T., Kuragano, M., Yamada, M. and Sugimoto, R. (2024)Comparing nearshore and embayment scale assessments of submarine groundwater discharge: Significance of offshore groundwater discharge as a nutrient pathway. Science of the Total Environment '''908''' 168068</ref>). | ||
+ | |||
+ | The investigation of subsurface geological structures by seismic profiling can be instructive to determine the geological origin of SGD and its flow paths. Geoelectrical methods are useful to determine the subsurface soil structure and groundwater salinity and distribution. Geoelectric instrumentation consist of an array of multiple electrodes, either directly inserted into the ground deployed on the sediment surface or, in some cases, towed behind a boat. For further details, see [[Groundwater management in low-lying coastal zones #Groundwater modelling and observation|Groundwater modelling and observation]]. | ||
==Impact on coastal water quality== | ==Impact on coastal water quality== | ||
+ | The study of Luijendijk et al. (2019<ref name=LGM/>), which is based on numerical modeling, reveals a high spatial variability of the coastal discharge of fresh groundwater and nutrients. For an estimated 26% (0.4%–39%) of the world’s estuaries, 17% (0.3%–31%) of the salt marshes and 14% (0.1–26%) of the coral reefs, the flux of terrestrial groundwater exceeds 25% of the river flux and poses a risk for pollution and eutrophication. However, estimates of SGD from tracer studies are substantially higher, suggesting that in many coastal zones fresh groundwater is a major nutrient supplier (Santos et al., 2021<ref>Santos, I.R., Chen, X., Lecher, A.L., Sawyer, A.H., Moosdorf, N., Rodellas, V., Tamborski, J., Cho, H-M., Dimova, N., Sugimoto, R., Bonaglia, S., Li, H., Hajati, M-C. and Li, L. 2021. Submarine groundwater discharge impacts on coastal nutrient biogeochemistry. Nat Rev Earth Environ 2: 307–323</ref>). | ||
+ | |||
+ | Nutrient input through submarine groundwater discharge (SGD) is assumed to play a significant role in nutrient cycling and primary productivity in the coastal ocean (Slomp and Van Cappellen, 2004<ref>Slomp, C.P. and Van Cappellen, P. (2004) Nutrient inputs to the coastal ocean through submarine groundwater discharge: controls and potential impact. J. Hydrol. '''295''' 64–86</ref>). The biogeochemistry of groundwater is generally more dominated by anoxic conditions than surface flow, in which case SGD has increased proportions of bioavailable ammonium. Although different biogeochemical processes can remove nitrogen, the N:P ratio in organic-rich groundwater is often higher than the Redfield ratio (16:1). This is due to net production of non-conservative nitrate through mineralization of organic matter and adsorption of non-conservative phosphorus (P) onto sediment (Robinson et al., 2018<ref name=R18>Robinson, C.E., Xin, P., Santos, I.R., Charette, M.A., Li, L. and Barry, D.A. (2018) Groundwater dynamics in subterranean estuaries of coastal unconfined aquifers: controls on submarine groundwater discharge and chemical inputs to the ocean. Adv. Water Resour. '''115''' 315–331</ref>). | ||
− | + | Benthic and water column primary production can be enhanced in outflow areas of submarine groundwater. For example, observations in intertidal groundwater outflow areas show changes in microbiological communities and proliferation of benthic animals, including mussels (Leitao et al., 2015<ref>Leitao F., Encarnaçao J., Range P., Schmelz R. M., Teodosio M. A. and Chicharo L. (2015). Submarine groundwater discharges create unique benthic communities in a coastal sandy marine environment. Estuar. Coast. Shelf Sci. '''163''' 93–98</ref>; Welti et al., 2015<ref>Welti N., Gale D., Hayes M., Kumar A., Gasparon M., Gibbes B. et al. (2015). Intertidal diatom communities reflect patchiness in groundwater discharge. Estuar. Coast. Shelf Sci. '''163''' 116–124</ref>; Taniguchi et al., 2019<ref name=T19/>). Conversely, excess nutrient loadings via polluted groundwater into coastal seas may cause eutrophication, harmful blooms and green tides (Cho et al., 2019<ref>Cho H. M., Kim G. and Shin K. H. (2019). Tracing nitrogen sources fueling coastal green tides off a volcanic island using radon and nitrogen isotopic tracers. Sci. Total Environ. '''665''' 913–919</ref>). | |
− | + | SGD does not only discharge nutrients, but also dissolved metals and pathogens. The effect is enhanced by the recirculated seawater which is enriched with [[pollutant]]s as it reacts with the aquifer sediment. Due to the long travel times of the groundwater, it can take decades for nutrients to be transported through the aquifer from their source to the ocean; the submarine groundwater discharge can therefore carry a long contamination history. This pollution legacy can be compounded with metal and organic contaminants that are mobilized from the sediment as a result of salt intrusion into the aquifer (Robinson et al., 2018<ref name=R18/>). | |
− | The [[pollutant]] concentration in the nearshore water and its impact on the chemistry and biology of this area | + | The [[pollutant]] concentration in the nearshore water and its impact on the chemistry and biology of this area depend not only on the [[pollutant]] fluxes but also on the intensity of mixing and the exchange with the open ocean. Thus, in order to determine whether SGD in an area of interest is of actual or probable importance, estimates must be determined of: |
* the magnitude of submarine groundwater discharge and | * the magnitude of submarine groundwater discharge and | ||
* the intensity of mixing in the nearshore sea area and the exchange between the nearshore sea and the open ocean. | * the intensity of mixing in the nearshore sea area and the exchange between the nearshore sea and the open ocean. | ||
==Methods for estimating the magnitude of SGD == | ==Methods for estimating the magnitude of SGD == | ||
− | + | Complementing several ongoing studies addressing the problem of submarine groundwater discharge estimation, an initiative was launched by the International Atomic Energy Agency (IAEA) and UNESCO in 2000 to assess the importance of SGD and the effectiveness of prediction methodologies for coastal zone management. The results of the projects undertaken in the framework of this initiative were presented in SCOR-LOICZ (2004) as well as in Burnett et al. (2006 <ref name="Burnett">Burnett, W.C., et al. (2006) Quantifying submarine groundwater discharge in the coastal zone via multiple methods. ''Sciences of the Total Environment'', '''367''' 498-543</ref>). A comprehensive report concerning current knowledge about SGD was presented by Gallardo and Marui (2006 <ref name="Gallardo">Gallardo A.H. and Marui A. (2006) Submarine groundwater discharge: an outlook of recent advances and current knowledge. ''Geo-Mar Lett'' ''26''' 102-113.</ref>). | |
− | Complementing several ongoing studies addressing the problem of submarine groundwater discharge estimation, an initiative was launched by the International Atomic Energy Agency (IAEA) and UNESCO in 2000 to assess the importance of SGD and the effectiveness of prediction methodologies for coastal zone management. The results of the projects undertaken in the framework of this initiative were presented in SCOR-LOICZ (2004) as well as in Burnett et al. (2006 <ref name="Burnett">Burnett, W.C., et al. (2006) Quantifying submarine groundwater discharge in the coastal zone via multiple methods. ''Sciences of the Total Environment'', '''367''' 498-543 | ||
The methods to estimate SGD can be distinguished in: | The methods to estimate SGD can be distinguished in: | ||
Line 45: | Line 51: | ||
===Model studies=== | ===Model studies=== | ||
+ | These investigations involve hydrogeological methods based on the water balance approach and numerical models. | ||
+ | |||
+ | In the water balance approach, the estimation of SGD is based on the water balance equation at the basin scale: | ||
− | + | ''Fresh water discharge = precipitation - evapotranspiration - surface discharge - groundwater storage''. | |
− | + | For extended periods (i.e. years), over which the change of water storage can be neglected, precipitation, evapotranspiration and surface discharge must be accurately known in order to reliably estimate SGD. The water balance approach only provides the fresh groundwater component of SGD as the total volume of groundwater discharging to the sea during the selected time period. Thus, the results of the water balance approach are not comparable with locally measured values or with results of numerical models that provide the distribution of SGD over the discharge area. An example of the water balance approach is the work of Sekulic and Vertacnik (1996 <ref name=SV>Sekulic, B. and Vertacnik, A. (1996) Balance of Average Annual Fresh Water Inflow into the Adriatic Sea. Water Resources Development, '''12'''(1) 89-97.</ref>), who estimated SGD in the Adriatic Sea. | |
Numerical models used in submarine groundwater discharge studies differ in the degree of complexity. Groundwater models neglecting density effects, as for instance MODFLOW <ref name="McDonalt">McDonalt M.G. and Harbaugh A.W. (1988) ''A modular three-dimensional finite-differences groundwater flow model''. US Geol Surv Open-File Report 83-875, 528p.</ref>, have been widely used. They provide only the freshwater component of SGD and can appropriately simulate the flow in the part of the coastal aquifer upstream of the saltwater intrusion area. SGD in such simulations is the groundwater flux at the seaward model boundary, assuming that there is no groundwater extraction in the area downstream of this boundary up to the shore. The collection of the data needed for the model calibration requires a considerable effort and it is time consuming, particularly for the parameters varying in time such as water levels and fluxes. However, in areas in which such data are available, these numerical models represent an efficient tool for the estimation of the freshwater part of SGD. | Numerical models used in submarine groundwater discharge studies differ in the degree of complexity. Groundwater models neglecting density effects, as for instance MODFLOW <ref name="McDonalt">McDonalt M.G. and Harbaugh A.W. (1988) ''A modular three-dimensional finite-differences groundwater flow model''. US Geol Surv Open-File Report 83-875, 528p.</ref>, have been widely used. They provide only the freshwater component of SGD and can appropriately simulate the flow in the part of the coastal aquifer upstream of the saltwater intrusion area. SGD in such simulations is the groundwater flux at the seaward model boundary, assuming that there is no groundwater extraction in the area downstream of this boundary up to the shore. The collection of the data needed for the model calibration requires a considerable effort and it is time consuming, particularly for the parameters varying in time such as water levels and fluxes. However, in areas in which such data are available, these numerical models represent an efficient tool for the estimation of the freshwater part of SGD. | ||
Line 54: | Line 63: | ||
More recently, numerical models considering density effects have been applied in SGD studies (an extensive review of such models can be found in Bear et al. 1999 <ref name="Bear">Bear J. et al. (Eds), (1999) ''Seawater Intrusion in Coastal Aquifers. Concepts, Methods and Practices''. Kluwer Academic Publishers, Dordrecht.</ref>). These models include the nearshore terrestrial part of the aquifer as well as the marine part below the sea floor, where density effects are relevant. They provide both the fresh groundwater discharge and the discharge of the seawater recirculated in the aquifer. Their application is feasible only if subsoil data are available. However, particularly for the part of the aquifer below the sea floor, the collection of such data is difficult. Therefore applications of such models, as those presented by Smith and Turner (2001 <ref name="Smith.">Smith A.J. and Turner J.V. (2001) Density-dependent surface water-groundwater interaction and nutrient discharge in the Swan-Canning Estuary. ''Hydrological Proc'', '''15''' 2595-2616.</ref>), Kaleris (2002 <ref name="Kaleris.">Kaleris V. et al. (2002) Modelling submarine groundwater discharge: an example from the western Baltic Sea. ''J. Hydrology'', '''265''' 76-99.</ref>), Langevin (2003 <ref name="Langevin">Langevin G.D. (2003) Simulation of Submarine Ground Water Discharge to a Marine Estuary: Biscayne Bay, Fl. ''Ground Water'', '''41'''(6) 758-771.</ref>) and Destouni and Prieto (2003 <ref name="Destouni">Destouni G. and Prieto C. (2003) On the possibility for generic modelling of submarine groundwater discharge. ''Biogeochemistry'' '''66''' 171-186.</ref>), are limited. | More recently, numerical models considering density effects have been applied in SGD studies (an extensive review of such models can be found in Bear et al. 1999 <ref name="Bear">Bear J. et al. (Eds), (1999) ''Seawater Intrusion in Coastal Aquifers. Concepts, Methods and Practices''. Kluwer Academic Publishers, Dordrecht.</ref>). These models include the nearshore terrestrial part of the aquifer as well as the marine part below the sea floor, where density effects are relevant. They provide both the fresh groundwater discharge and the discharge of the seawater recirculated in the aquifer. Their application is feasible only if subsoil data are available. However, particularly for the part of the aquifer below the sea floor, the collection of such data is difficult. Therefore applications of such models, as those presented by Smith and Turner (2001 <ref name="Smith.">Smith A.J. and Turner J.V. (2001) Density-dependent surface water-groundwater interaction and nutrient discharge in the Swan-Canning Estuary. ''Hydrological Proc'', '''15''' 2595-2616.</ref>), Kaleris (2002 <ref name="Kaleris.">Kaleris V. et al. (2002) Modelling submarine groundwater discharge: an example from the western Baltic Sea. ''J. Hydrology'', '''265''' 76-99.</ref>), Langevin (2003 <ref name="Langevin">Langevin G.D. (2003) Simulation of Submarine Ground Water Discharge to a Marine Estuary: Biscayne Bay, Fl. ''Ground Water'', '''41'''(6) 758-771.</ref>) and Destouni and Prieto (2003 <ref name="Destouni">Destouni G. and Prieto C. (2003) On the possibility for generic modelling of submarine groundwater discharge. ''Biogeochemistry'' '''66''' 171-186.</ref>), are limited. | ||
− | Luijendijk et al. (2020<ref name=LGM/>) simulated submarine and terrestrial groundwater discharge in coastal groundwater systems using a numerical model of coupled density-driven groundwater flow and solute transport that solved the fluid flow and solute transport equations and the equations of state for fluid density and viscosity in a two-dimensional cross section of the subsurface. The model simulated the flow of fresh (meteoric) groundwater, the mixing and recirculation of seawater at the fresh-salt water interface and the onshore and offshore discharge of groundwater. Assumed were a constant linear slope and a fully saturated standard aquifer of 100 m thickness, which is roughly equal to the thickness where globally the majority of young groundwater and active groundwater circulates. For the global model, 40,082 coastal watersheds were selected using global watershed and coastline datasets. Permeability of each coastal watershed was extracted from a global dataset of near-surface permeability. Comparison between modeled and measured average hydraulic gradients in 336 coastal watersheds showed that the model provides a realistic estimate of coastal groundwater flow. The model outcome for the global fresh submarine groundwater discharge is lower than estimates of earlier studies. The authors explain why the fresh submarine groundwater flow in previous studies is often overestimated; this is the case, for example, when a large part of the overall groundwater recharge is not discharged by SGD but by | + | Luijendijk et al. (2020<ref name=LGM/>) simulated submarine and terrestrial groundwater discharge in coastal groundwater systems using a numerical model of coupled density-driven groundwater flow and solute transport that solved the fluid flow and solute transport equations and the equations of state for fluid density and viscosity in a two-dimensional cross section of the subsurface. The model simulated the flow of fresh (meteoric) groundwater, the mixing and recirculation of seawater at the fresh-salt water interface and the onshore and offshore discharge of groundwater. Assumed were a constant linear slope and a fully saturated standard aquifer of 100 m thickness, which is roughly equal to the thickness where globally the majority of young groundwater and active groundwater circulates. For the global model, 40,082 coastal watersheds were selected using global watershed and coastline datasets. Permeability of each coastal watershed was extracted from a global dataset of near-surface permeability. Comparison between modeled and measured average hydraulic gradients in 336 coastal watersheds showed that the model provides a realistic estimate of coastal groundwater flow. The model outcome for the global fresh submarine groundwater discharge is lower than estimates of earlier studies. The authors explain why the fresh submarine groundwater flow in previous studies is often overestimated; this is the case, for example, when a large part of the overall groundwater recharge is not discharged by SGD but by surface flows. |
+ | |||
+ | Aquifer systems are usually heterogeneous, and it is difficult to obtain sufficient representative values such as hydraulic conductivity and porosity to adequately characterize this heterogeneity. Hydraulic conductivity often varies over several orders of magnitude within short distances. Aquifer thickness and hydraulic conductivity values are the most important factors for reliable estimates of groundwater seepage rates by theoretical and numerical analysis<ref name="Burnett"/>. | ||
===Experimental studies=== | ===Experimental studies=== | ||
− | The most important experimental methods for | + | [[File:GroundwaterTracer.jpg|thumb|right|300px|Fig. 3. Determination of the SGD (fresh+salt) by measuring tracer concentrations <math>c(x)</math> and using the balance equation explained in the figure. Assumptions: the tracer is well mixed over the vertical, the system is laterally uniform. Symbols: <math>C_{gw}=</math> concentration of a tracer representative of groundwater, <math>U_{gw}=</math> SGD per unit soil surface, <math>u=</math> water flow velocity, <math>K=</math> horizontal dispersion coefficient, <math>h=</math> water depth. The fresh groundwater discharge can be found from the salinity balance.]] |
+ | |||
+ | The most important experimental methods for investigation of submarine groundwater discharge are seepage measuring techniques and the use of geochemical tracers such as [[radium]] [[isotopes]] and [[radon]], which are both enriched in groundwater relative to seawater. | ||
− | An overview of different types of seepage meters used to measure SGD at the bottom of the sea, as well as conclusions concerning their applicability in the field, have been presented by Taniguchi et al. (2003 <ref name="Taniguchi">Taniguchi M. et al. (2003) Spatial and temporal distribution of submarine groundwater discharge rates obtained | + | An overview of different types of seepage meters used to measure SGD at the bottom of the sea, as well as conclusions concerning their applicability in the field, have been presented by Taniguchi et al. (2003 <ref name="Taniguchi">Taniguchi M. et al. (2003) Spatial and temporal distribution of submarine groundwater discharge rates obtained from various types of seepage meters at a site in the northeastern Gulf of Mexico. ''Biogeochemistry'' '''66''' 35-53</ref> and 2019<ref name=T19/>). Seepage meters provide local SGD values because of the variability of the hydraulic properties of the sea bottom material and the relatively small area (usually <<1m<sup>2</sup>), over which SGD is measured. Taking into consideration the variability of SGD in time, it can be concluded that in order to obtain representative values of SGD for a shore, which can be a few kilometres long, a large number of measurements at different locations and at different times must be performed. The results of seepage meter applications and comparisons of various methods have been presented by Burnett et al. (2006 <ref name="Burnett">Burnett, W.C., et al. (2006) Quantifying submarine groundwater discharge in the coastal zone via multiple methods. ''Sciences of the Total Environment'', '''367''' 498-543.</ref>). Another measuring tool is described in [[Submarine groundwater discharge in coastal regions: development of a new sampling technique]]. |
− | [[Radon]] is produced by the decay of radioactive [[isotopes]] in the sediments. It is therefore strongly enriched both in the freshwater part as well as in the recirculated part of submarine groundwater discharge relative to coastal marine waters <ref name="Oberdorfer">Oberdorfer J.A. (2003) Hydrogeologic modeling of submarine groundwater discharge: comparison to other quantitative methods. ''Biogeochemistry'' '''66''' 159-169.</ref>. | + | The balance equation that enables the determination of the submarine groundwater discharge (the sum of fresh groundwater and recirculated seawater) from tracer measurements (e.g. Radon isotope) is shown in Fig. 3. The tracer element [[Radon]] (<sup>222</sup>Rn, half time = 3.8 days) is produced by the decay of radioactive [[isotopes]] in the sediments. It is therefore strongly enriched both in the freshwater part as well as in the recirculated part of submarine groundwater discharge relative to coastal marine waters <ref name="Oberdorfer">Oberdorfer J.A. (2003) Hydrogeologic modeling of submarine groundwater discharge: comparison to other quantitative methods. ''Biogeochemistry'' '''66''' 159-169.</ref>. Automated measurement methods allow for easy time-series and spatial survey measurements. However, freshwater and recirculated parts of SGD can hardly be distinguished through [[radon]] measurements. Nearshore water samples used to measure [[radon]] activity, do not always provide values that are representative for the whole water column. An important factor is the degree of mixing of SGD with coastal surface water. <sup>222</sup>Rn can escape to the atmosphere; a corresponding additional term must be therefore included in the balance equation of Fig. 3. |
− | [[Radium]] is | + | The tracer element [[Radium]] is adsorbed onto the surface of geologic material and is immobile in fresh groundwater. When saltwater intrudes the aquifer and replaces freshwater in the pores, radium is desorbed from sediment and becomes mobile (Moore, 1996<ref name="Moore">Moore W.S. (1996) Large groundwater inputs to coastal waters revealed by <sup>226</sup>Ra enrichment. ''Nature'', '''380''' 612-614</ref>). Thus, [[radium]] is transferred to the nearshore seawater primarily by the recirculated seawater involved in saltwater intrusion (Oberdorfer, 2003<ref name="Oberdorfer">Oberdorfer J.A. (2003) Hydrogeologic modeling of submarine groundwater discharge: comparison to other quantitative methods. ''Biogeochemistry'' '''66''' 159-169</ref>). Since this water discharges into the sea mixed with the fresh groundwater, it is not possible, as in case of [[radon]], to distinguish the portions of fresh groundwater and seawater in SGD. |
− | + | Several other natural radioactive (<sup>3</sup>H, <sup>14</sup>C, U isotopes, etc.) and stable (<sup>2</sup>H, <sup>3</sup>He, <sup>4</sup>He, <sup>13</sup>C, <sup>15</sup>N, <sup>18</sup>O, <sup>87/88</sup>Sr, etc.) isotopes and some anthropogenic atmospheric gases (e.g., CFC's) have been used for conducting SGD investigations, tracing water masses, and calculating the age of groundwater<ref name="Burnett"/>. | |
− | An overview of the technologies developed for the measurement of [[radon]] and [[radium]] | + | Kwon et al. (2014<ref name=Kwon/>) determined the submarine groundwater discharge from a global compilation of <sup>228</sup>Ra observations, by using an inverse model. They found that the SGD integrated over the Atlantic and Indo-Pacific Oceans between 60°S and 70°N is (12 ± 3).10<sup>4</sup> km<sup>3</sup>/yr, which is 3 to 4 times greater than the freshwater fluxes into the oceans by rivers. Although this discharge consists mainly of recirculating seawater, it is enriched during its temporary invasion of the coastal aquifer with trace elements, rare earth elements, nutrients, and dissolved inorganic and organic carbon through biogeochemical reactions. This suggests that SGD is an important pathway for dissolved terrestrial materials to the coastal waters. |
+ | |||
+ | An overview of the technologies developed for the measurement of [[radon]] and [[radium]], the limitations of the methods as well as applications for the estimation of submarine groundwater discharge, can be found in Burnett et al. (2006 <ref name="Burnett">Burnett, W.C., et al. (2006) Quantifying submarine groundwater discharge in the coastal zone via multiple methods. ''Sciences of the Total Environment'', '''367''' 498-543.</ref>). | ||
===Indirect indicators of SGD=== | ===Indirect indicators of SGD=== | ||
+ | Application of the methods described above involves considerable financial, labor and/or computational effort. Thus, before such methods are applied in coastal zone management studies, it is important to determine if the magnitude of submarine groundwater discharge is relevant. Results of investigations presented in the literature can hardly help in such assessments as they vary widely. High seepage values (above 100 cm per day and unit outflow area) as well as low values (below 5 cm per day and unit outflow area) have been observed Burnett et al. (2006 <ref name="Burnett">Burnett, W.C., et al. (2006) Quantifying submarine groundwater discharge in the coastal zone via multiple methods. ''Sciences of the Total Environment'', '''367''' 498-543.</ref>). A way to determine locations of SGD and the importance of SGD in an area, is to use indirect indicators (SCOR-LOIZ, 2004<ref name="SCOR-LOIZ ">SCOR-LOIZ (2004) ''Submarine groundwater discharge management implications, measurements and effects''. IHP-VI Series on Groundwater 5. IOC Manuals and Guides 44. United Nations Educational, Scientific and Cultural Organization, Paris</ref>; Taniguchi, 2019<ref name=T19/>). Hotspots of submarine groundwater discharge can reveal themselves in several ways. The color, temperature, salinity, or some other geochemical fingerprint might distinguish the water itself. Other clues include<ref name="Burnett"/>: | ||
+ | *The groundwater escaping from the sea bottom might be coloured by tiny gas bubbles. | ||
+ | *Surrounding sediment might be stained red by the oxidation of iron. | ||
+ | *There are cold-water anomalies in the open water during the summer and warm-water anomalies in winter as well as salinity anomalies; plumes of buoyant low-density (fresh/brackish) groundwater can be detected with digital thermal infrared cameras mounted on light aircraft or on drones. The typical temperature resolution is about 0.1 <sup>o</sup>C and the spatial resolution about 0.5 m. Salinity anomalies have long been used to identify subsea freshwater seeps. | ||
+ | *Growth of freshwater coastal vegetation. | ||
+ | *Freezing of the sea floor (so-called 'anchor ice') while the saline open waters of the bay are still ice-free. | ||
+ | *Ice-free spots (so-called 'wind-spots') above submarine groundwater outflow containing relatively warm freshwater. | ||
+ | *The presence of coastal ponds or unconsolidated coastal bluffs, which may maintain a high hydraulic head near shore. | ||
+ | *Barite, oxidized shells, or beach rock may indicate the occurrence of groundwater discharges. | ||
+ | *Pockmarks in the fine-grained sediments of the sea floor have been identified as bathymetric expressions of groundwater seeps (Fig. 2). | ||
+ | *Water itself can be domed and 'boiling' If the submarine groundwater outflow is great enough. | ||
+ | * The levels of [[radium]], [[radon]], methane, hydrogen sulphide or carbon dioxide might be elevated. | ||
+ | * Stable nitrogen isotope ratios (<math>\delta^{15}</math>N) of primary producers can be useful in detecting the direct linkage between groundwater inputs and marine organisms when the dissolved inorganic nitrogen (DIN) of groundwater is isotopically distinct from other DIN sources. | ||
− | |||
− | |||
− | |||
− | |||
− | |||
==Mixing in the nearshore sea== | ==Mixing in the nearshore sea== | ||
− | |||
The concentration of [[pollutant|pollutants]], transferred to the nearshore sea area by submarine groundwater discharge, depends on the intensity of mixing in this area, which is due to hydrodynamic circulation, transport and [[dispersion]]. The estimation of the [[pollutant]] concentration in the nearshore sea using numerical models, which simulate the forementioned processes, is computationally intensive and requires data, which in many cases are not readily available. A more convenient approach is to use cell models (well-mixed reservoir models). | The concentration of [[pollutant|pollutants]], transferred to the nearshore sea area by submarine groundwater discharge, depends on the intensity of mixing in this area, which is due to hydrodynamic circulation, transport and [[dispersion]]. The estimation of the [[pollutant]] concentration in the nearshore sea using numerical models, which simulate the forementioned processes, is computationally intensive and requires data, which in many cases are not readily available. A more convenient approach is to use cell models (well-mixed reservoir models). | ||
− | An application of a cell model is given by Uchiyama et al. (2000 <ref name="Uchiyama">Uchiyama Y., Nadaoka K., Roelke P., Adachi K. and Yagi H. (2000) Submarine groundwater discharge into the sea and associated nutrient transport in a sandy beach. ''Water Resources Res.'' '''36'''(6) 1467-1479.</ref>), who investigated the nutrient concentration in the nearshore sea due to SGD. They found that the long-term [[pollutant]] concentration depends on the relationship between the [[pollutant]] fluxes due to SGD and the exchange flux between the nearshore sea and the open ocean. For the conditions of the investigated case, these authors found that the volumetric exchange flux between the nearshore sea and the open ocean is three orders of magnitude larger than the SGD flux. Thus, the long term [[pollutant]] concentrations, resulting under the assumption that the [[pollution]] of the open ocean water is negligible, were found to be of minor importance for the nearshore sea environment. | + | An application of a cell model is given by Uchiyama et al. (2000 <ref name="Uchiyama">Uchiyama Y., Nadaoka K., Roelke P., Adachi K. and Yagi H. (2000) Submarine groundwater discharge into the sea and associated nutrient transport in a sandy beach. ''Water Resources Res.'' '''36'''(6) 1467-1479.</ref>), who investigated the nutrient concentration in the nearshore sea due to SGD. They found that the long-term [[pollutant]] concentration depends on the relationship between the [[pollutant]] fluxes due to SGD and the exchange flux between the nearshore sea and the open ocean. For the conditions of the investigated case, these authors further found that the volumetric exchange flux between the nearshore sea and the open ocean is three orders of magnitude larger than the SGD flux. Thus, the long term [[pollutant]] concentrations, resulting under the assumption that the [[pollution]] of the open ocean water is negligible, were found to be of minor importance for the nearshore sea environment. |
− | For estimations of the exchange of water between the nearshore sea (surf zone) and the offshore zone, a relatively simple model presented by Inman et al. (1971 <ref name="Inman">Inman D.L., | + | For estimations of the exchange of water between the nearshore sea (surf zone) and the offshore zone, a relatively simple model presented by Inman et al. (1971 <ref name="Inman">Inman D.L., Tait R.J. and Nordstrom C.E. (1971) Mixing in the surf zone. ''J. Geophys. Res.'', '''76''' 3493-3514.</ref>) can be used. The data required are the wave and [[Characteristics of sedimentary shores|beach characteristics]] (breaker height, breaker angle, beach slope in the surf zone, beach slope offshore). For open beaches, typical wave and beach characteristics, it is probable that the water exchange between the surf zone and the offshore strongly dilutes [[pollutant|pollutants]] transported in the surf zone by submarine groundwater discharge, for usual values of SGD (5-50 cm/day) as reported in Burnett et al. (2006 <ref name="Burnett">Burnett, W.C., et al. (2006) Quantifying submarine groundwater discharge in the coastal zone via multiple methods. ''Sciences of the Total Environment'', '''367''' 498-543.</ref>), Gallardo and Marui (2006 <ref name="Gallardo">Gallardo A.H. and Marui A. (2006) Submarine groundwater discharge: an outlook of recent advances and current knowledge. ''Geo-Mar Letters'' ''26''' 102-113.</ref>) and Gilfedder et al. (2021<ref>Gilfedder B.S., Waska H., Wismeth F. and Frei S. (2021) Using heat as a tracer to map and quantify water infiltration and exfiltration along a complex high energy beach face. Estuarine, Coastal and Shelf Science '''250''' 107140</ref>). However, in semi-closed bays or in nearshore seas with weak wave action and circulation, it can be shown using cell models, that the long term [[pollutant]] concentration in the nearshore environment due to the impact of SGD is not negligible. In such cases, measures to reduce the [[pollutant]] load of groundwater are required. |
Latest revision as of 14:53, 20 September 2024
Submarine groundwater discharge (SGD) can have a significant influence on the coastal environment. This article examines the underlying processes and the importance of submarine groundwater discharge for coastal zone management. SGD is the discharge of groundwater going directly into the sea, and not via surface streams. Groundwater is synonymous with soil pore water. In order to assess the magnitude of SGD, different investigations based on models and on direct measurements have been carried out. Indirect indicators of SGD are also considered.
Contents
Process description and estimates
Submarine groundwater discharge (SGD) is defined as the discharging flow out of the aquifer across the sea floor [1]. It is a process complementary to saltwater intrusion, which is defined as the invasion of seawater at the lower part of the aquifer due to the density difference between fresh groundwater and seawater, see Groundwater management in low-lying coastal zones. The processes are shown schematically in Figures 1a and 1b.
If mixing between fresh groundwater and the seawater wedge is weak, it can be assumed that the interface between the two areas is sharp (Figure 1a). In such cases, the submarine fresh groundwater flows to the sea above the saltwater wedge. During the winter period the interface is located more landward than in summer, assuming that the groundwater levels occurring in fall are relatively low. During summer, the interface moves seaward, assuming a rise of the groundwater level during spring, while, simultaneously with fresh groundwater discharge, seawater outflow takes place from the saltwater wedge. The seawater outflow is generally much greater than the fresh groundwater discharge[2].
In general there is no sharp interface between the freshwater and the saltwater. Due to dispersion and molecular diffusion a freshwater-seawater mixing zone is built between the two areas. The mixing causes the salt water in the deeper part of the aquifer to become lighter, rise up and discharge back to the sea (Figure 1b). In this case, SGD consists of a mixture of fresh groundwater and recirculated seawater. The recirculation of seawater, in the nearshore part of the aquifer, is further enhanced by mechanisms acting from the marine side such as tidal pumping and wave set-up. The time variation of SGD exhibits components at small time scales (minutes, hours, days) as well as components of large time scale, which are due to the seasonal motion of the mixing zone and large-scale sea level variations. Because aquifer recharge is faster than aquifer discharge, seawater infiltration by waves and tides creates an over-height of the groundwater table (pumping effect). This over-height can exceed, during storm conditions, all other geophysical drivers of fluid flow across the sediment-water interface (Sawyer et al., 2013[3]).
Concerning the contribution of the fresh groundwater flow and the seawater recirculation to the total SGD, it is estimated that:
- The global total SGD flux, which includes recirculated seawater, could be three to four times larger than the global river flux, based on measured radium isotope concentrations in seawater (Kwon et al., 2014[4].) The terrestrially derived SGD (fresh water) was estimated by Burnett et al. (2003[5]) in the range of 6-10% of the surface waters discharging into the ocean. However, more recent calculations by Luijendijk et al. (2020[6]) show that the submarine groundwater discharge of terrestrial origin cannot exceed 5.5% of the river input into the world’s oceans, assuming that all groundwater recharge in coastal watersheds were to discharge directly into the oceans. Sawyer et al. (2016[7]) estimate that the total volumetric rate of fresh SGD from the contiguous United States to the oceans may amount to 25 ± 7 cubic km/year, or 1-2% of surface runoff.
- Assuming that recirculation is only due to density effects and mixing, recirculated seawater can be up to 70% of the SGD (Smith, 2004[8]; Kaleris, 2006[9]);
- Assuming that recirculation is also due to wave setup and tide, the recirculated sea water may constitute up to 96% of the SGD (Li et al. 1999[10]). However, Luijendijk et al.[6] estimate that the fresh SGD is even a much smaller fraction, 0.06% (0.0003%–0.2%), of the global total SGD flux, which thus consists almost entirely of recirculated seawater. According to these authors, the contribution of fresh groundwater discharge to the oceans is globally in the order of ~0.6% (0.004%–1.3%) of the total freshwater input and ~2% (0.003%–7.7%) of the solute input for carbon, nitrogen, silica and strontium.
Submarine groundwater pathways
Submarine groundwater flow is usually three dimensional and highly complex due to the patchy nature of burrows, subtle geomorphological gradients, and heterogeneous sediments and vegetation. It is distributed over large areas, but particularly strong, concentrated, submarine groundwater discharges occur in case of karstic aquifers discharging into the sea (karstic submarine springs). Fault lines or fracture patterns and submarine paleochannels, infilled with permeable sediments, are also preferential pathways of SGD (Taniguchi et al., 2019[11]). Submarine groundwater discharge can take place not only near the shore but also at great offshore distances. The latter occurs when confined aquifers, underneath the continental shelf, have their outcrop on the sea bottom and at a large distance from the shore. Concentrated outflows at the sea bottom occur in cases where the outcrops of alluvial aquifers are of limited area. Such outflow locations on the sea bottom sometimes form pockmarks in the fine-grained sediments of the sea floor, see Fig. 2.
Coastal groundwater discharge hotspots cover 9% (0.02–30)% of the global coastline and are predominantly located in areas with a steep coastal topography due to glacio-isostatic rebound, active tectonics or volcanic activity and areas consisting of permeable unconsolidated sediments, carbonates or volcanic rocks. The distribution of hotspots is consistent with documented sites of high fresh groundwater discharge globally that are predominantly located in North America, Europe, and East Asia (Luijendijk et al., 2019[6]). A list of SGD hotspots from field studies reported in the literature is presented in Nakajima et al. (2024[12]).
The investigation of subsurface geological structures by seismic profiling can be instructive to determine the geological origin of SGD and its flow paths. Geoelectrical methods are useful to determine the subsurface soil structure and groundwater salinity and distribution. Geoelectric instrumentation consist of an array of multiple electrodes, either directly inserted into the ground deployed on the sediment surface or, in some cases, towed behind a boat. For further details, see Groundwater modelling and observation.
Impact on coastal water quality
The study of Luijendijk et al. (2019[6]), which is based on numerical modeling, reveals a high spatial variability of the coastal discharge of fresh groundwater and nutrients. For an estimated 26% (0.4%–39%) of the world’s estuaries, 17% (0.3%–31%) of the salt marshes and 14% (0.1–26%) of the coral reefs, the flux of terrestrial groundwater exceeds 25% of the river flux and poses a risk for pollution and eutrophication. However, estimates of SGD from tracer studies are substantially higher, suggesting that in many coastal zones fresh groundwater is a major nutrient supplier (Santos et al., 2021[13]).
Nutrient input through submarine groundwater discharge (SGD) is assumed to play a significant role in nutrient cycling and primary productivity in the coastal ocean (Slomp and Van Cappellen, 2004[14]). The biogeochemistry of groundwater is generally more dominated by anoxic conditions than surface flow, in which case SGD has increased proportions of bioavailable ammonium. Although different biogeochemical processes can remove nitrogen, the N:P ratio in organic-rich groundwater is often higher than the Redfield ratio (16:1). This is due to net production of non-conservative nitrate through mineralization of organic matter and adsorption of non-conservative phosphorus (P) onto sediment (Robinson et al., 2018[15]).
Benthic and water column primary production can be enhanced in outflow areas of submarine groundwater. For example, observations in intertidal groundwater outflow areas show changes in microbiological communities and proliferation of benthic animals, including mussels (Leitao et al., 2015[16]; Welti et al., 2015[17]; Taniguchi et al., 2019[11]). Conversely, excess nutrient loadings via polluted groundwater into coastal seas may cause eutrophication, harmful blooms and green tides (Cho et al., 2019[18]).
SGD does not only discharge nutrients, but also dissolved metals and pathogens. The effect is enhanced by the recirculated seawater which is enriched with pollutants as it reacts with the aquifer sediment. Due to the long travel times of the groundwater, it can take decades for nutrients to be transported through the aquifer from their source to the ocean; the submarine groundwater discharge can therefore carry a long contamination history. This pollution legacy can be compounded with metal and organic contaminants that are mobilized from the sediment as a result of salt intrusion into the aquifer (Robinson et al., 2018[15]).
The pollutant concentration in the nearshore water and its impact on the chemistry and biology of this area depend not only on the pollutant fluxes but also on the intensity of mixing and the exchange with the open ocean. Thus, in order to determine whether SGD in an area of interest is of actual or probable importance, estimates must be determined of:
- the magnitude of submarine groundwater discharge and
- the intensity of mixing in the nearshore sea area and the exchange between the nearshore sea and the open ocean.
Methods for estimating the magnitude of SGD
Complementing several ongoing studies addressing the problem of submarine groundwater discharge estimation, an initiative was launched by the International Atomic Energy Agency (IAEA) and UNESCO in 2000 to assess the importance of SGD and the effectiveness of prediction methodologies for coastal zone management. The results of the projects undertaken in the framework of this initiative were presented in SCOR-LOICZ (2004) as well as in Burnett et al. (2006 [1]). A comprehensive report concerning current knowledge about SGD was presented by Gallardo and Marui (2006 [19]).
The methods to estimate SGD can be distinguished in:
- (a) model investigations and
- (b) experimental investigations.
Model studies
These investigations involve hydrogeological methods based on the water balance approach and numerical models.
In the water balance approach, the estimation of SGD is based on the water balance equation at the basin scale:
Fresh water discharge = precipitation - evapotranspiration - surface discharge - groundwater storage.
For extended periods (i.e. years), over which the change of water storage can be neglected, precipitation, evapotranspiration and surface discharge must be accurately known in order to reliably estimate SGD. The water balance approach only provides the fresh groundwater component of SGD as the total volume of groundwater discharging to the sea during the selected time period. Thus, the results of the water balance approach are not comparable with locally measured values or with results of numerical models that provide the distribution of SGD over the discharge area. An example of the water balance approach is the work of Sekulic and Vertacnik (1996 [20]), who estimated SGD in the Adriatic Sea.
Numerical models used in submarine groundwater discharge studies differ in the degree of complexity. Groundwater models neglecting density effects, as for instance MODFLOW [21], have been widely used. They provide only the freshwater component of SGD and can appropriately simulate the flow in the part of the coastal aquifer upstream of the saltwater intrusion area. SGD in such simulations is the groundwater flux at the seaward model boundary, assuming that there is no groundwater extraction in the area downstream of this boundary up to the shore. The collection of the data needed for the model calibration requires a considerable effort and it is time consuming, particularly for the parameters varying in time such as water levels and fluxes. However, in areas in which such data are available, these numerical models represent an efficient tool for the estimation of the freshwater part of SGD.
More recently, numerical models considering density effects have been applied in SGD studies (an extensive review of such models can be found in Bear et al. 1999 [22]). These models include the nearshore terrestrial part of the aquifer as well as the marine part below the sea floor, where density effects are relevant. They provide both the fresh groundwater discharge and the discharge of the seawater recirculated in the aquifer. Their application is feasible only if subsoil data are available. However, particularly for the part of the aquifer below the sea floor, the collection of such data is difficult. Therefore applications of such models, as those presented by Smith and Turner (2001 [23]), Kaleris (2002 [24]), Langevin (2003 [25]) and Destouni and Prieto (2003 [26]), are limited.
Luijendijk et al. (2020[6]) simulated submarine and terrestrial groundwater discharge in coastal groundwater systems using a numerical model of coupled density-driven groundwater flow and solute transport that solved the fluid flow and solute transport equations and the equations of state for fluid density and viscosity in a two-dimensional cross section of the subsurface. The model simulated the flow of fresh (meteoric) groundwater, the mixing and recirculation of seawater at the fresh-salt water interface and the onshore and offshore discharge of groundwater. Assumed were a constant linear slope and a fully saturated standard aquifer of 100 m thickness, which is roughly equal to the thickness where globally the majority of young groundwater and active groundwater circulates. For the global model, 40,082 coastal watersheds were selected using global watershed and coastline datasets. Permeability of each coastal watershed was extracted from a global dataset of near-surface permeability. Comparison between modeled and measured average hydraulic gradients in 336 coastal watersheds showed that the model provides a realistic estimate of coastal groundwater flow. The model outcome for the global fresh submarine groundwater discharge is lower than estimates of earlier studies. The authors explain why the fresh submarine groundwater flow in previous studies is often overestimated; this is the case, for example, when a large part of the overall groundwater recharge is not discharged by SGD but by surface flows.
Aquifer systems are usually heterogeneous, and it is difficult to obtain sufficient representative values such as hydraulic conductivity and porosity to adequately characterize this heterogeneity. Hydraulic conductivity often varies over several orders of magnitude within short distances. Aquifer thickness and hydraulic conductivity values are the most important factors for reliable estimates of groundwater seepage rates by theoretical and numerical analysis[1].
Experimental studies
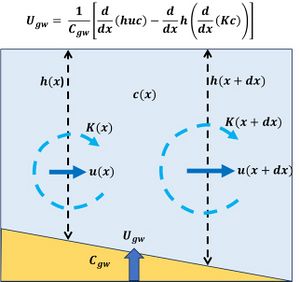
The most important experimental methods for investigation of submarine groundwater discharge are seepage measuring techniques and the use of geochemical tracers such as radium isotopes and radon, which are both enriched in groundwater relative to seawater.
An overview of different types of seepage meters used to measure SGD at the bottom of the sea, as well as conclusions concerning their applicability in the field, have been presented by Taniguchi et al. (2003 [27] and 2019[11]). Seepage meters provide local SGD values because of the variability of the hydraulic properties of the sea bottom material and the relatively small area (usually <<1m2), over which SGD is measured. Taking into consideration the variability of SGD in time, it can be concluded that in order to obtain representative values of SGD for a shore, which can be a few kilometres long, a large number of measurements at different locations and at different times must be performed. The results of seepage meter applications and comparisons of various methods have been presented by Burnett et al. (2006 [1]). Another measuring tool is described in Submarine groundwater discharge in coastal regions: development of a new sampling technique.
The balance equation that enables the determination of the submarine groundwater discharge (the sum of fresh groundwater and recirculated seawater) from tracer measurements (e.g. Radon isotope) is shown in Fig. 3. The tracer element Radon (222Rn, half time = 3.8 days) is produced by the decay of radioactive isotopes in the sediments. It is therefore strongly enriched both in the freshwater part as well as in the recirculated part of submarine groundwater discharge relative to coastal marine waters [28]. Automated measurement methods allow for easy time-series and spatial survey measurements. However, freshwater and recirculated parts of SGD can hardly be distinguished through radon measurements. Nearshore water samples used to measure radon activity, do not always provide values that are representative for the whole water column. An important factor is the degree of mixing of SGD with coastal surface water. 222Rn can escape to the atmosphere; a corresponding additional term must be therefore included in the balance equation of Fig. 3.
The tracer element Radium is adsorbed onto the surface of geologic material and is immobile in fresh groundwater. When saltwater intrudes the aquifer and replaces freshwater in the pores, radium is desorbed from sediment and becomes mobile (Moore, 1996[29]). Thus, radium is transferred to the nearshore seawater primarily by the recirculated seawater involved in saltwater intrusion (Oberdorfer, 2003[28]). Since this water discharges into the sea mixed with the fresh groundwater, it is not possible, as in case of radon, to distinguish the portions of fresh groundwater and seawater in SGD.
Several other natural radioactive (3H, 14C, U isotopes, etc.) and stable (2H, 3He, 4He, 13C, 15N, 18O, 87/88Sr, etc.) isotopes and some anthropogenic atmospheric gases (e.g., CFC's) have been used for conducting SGD investigations, tracing water masses, and calculating the age of groundwater[1].
Kwon et al. (2014[4]) determined the submarine groundwater discharge from a global compilation of 228Ra observations, by using an inverse model. They found that the SGD integrated over the Atlantic and Indo-Pacific Oceans between 60°S and 70°N is (12 ± 3).104 km3/yr, which is 3 to 4 times greater than the freshwater fluxes into the oceans by rivers. Although this discharge consists mainly of recirculating seawater, it is enriched during its temporary invasion of the coastal aquifer with trace elements, rare earth elements, nutrients, and dissolved inorganic and organic carbon through biogeochemical reactions. This suggests that SGD is an important pathway for dissolved terrestrial materials to the coastal waters.
An overview of the technologies developed for the measurement of radon and radium, the limitations of the methods as well as applications for the estimation of submarine groundwater discharge, can be found in Burnett et al. (2006 [1]).
Indirect indicators of SGD
Application of the methods described above involves considerable financial, labor and/or computational effort. Thus, before such methods are applied in coastal zone management studies, it is important to determine if the magnitude of submarine groundwater discharge is relevant. Results of investigations presented in the literature can hardly help in such assessments as they vary widely. High seepage values (above 100 cm per day and unit outflow area) as well as low values (below 5 cm per day and unit outflow area) have been observed Burnett et al. (2006 [1]). A way to determine locations of SGD and the importance of SGD in an area, is to use indirect indicators (SCOR-LOIZ, 2004[30]; Taniguchi, 2019[11]). Hotspots of submarine groundwater discharge can reveal themselves in several ways. The color, temperature, salinity, or some other geochemical fingerprint might distinguish the water itself. Other clues include[1]:
- The groundwater escaping from the sea bottom might be coloured by tiny gas bubbles.
- Surrounding sediment might be stained red by the oxidation of iron.
- There are cold-water anomalies in the open water during the summer and warm-water anomalies in winter as well as salinity anomalies; plumes of buoyant low-density (fresh/brackish) groundwater can be detected with digital thermal infrared cameras mounted on light aircraft or on drones. The typical temperature resolution is about 0.1 oC and the spatial resolution about 0.5 m. Salinity anomalies have long been used to identify subsea freshwater seeps.
- Growth of freshwater coastal vegetation.
- Freezing of the sea floor (so-called 'anchor ice') while the saline open waters of the bay are still ice-free.
- Ice-free spots (so-called 'wind-spots') above submarine groundwater outflow containing relatively warm freshwater.
- The presence of coastal ponds or unconsolidated coastal bluffs, which may maintain a high hydraulic head near shore.
- Barite, oxidized shells, or beach rock may indicate the occurrence of groundwater discharges.
- Pockmarks in the fine-grained sediments of the sea floor have been identified as bathymetric expressions of groundwater seeps (Fig. 2).
- Water itself can be domed and 'boiling' If the submarine groundwater outflow is great enough.
- The levels of radium, radon, methane, hydrogen sulphide or carbon dioxide might be elevated.
- Stable nitrogen isotope ratios ([math]\delta^{15}[/math]N) of primary producers can be useful in detecting the direct linkage between groundwater inputs and marine organisms when the dissolved inorganic nitrogen (DIN) of groundwater is isotopically distinct from other DIN sources.
Mixing in the nearshore sea
The concentration of pollutants, transferred to the nearshore sea area by submarine groundwater discharge, depends on the intensity of mixing in this area, which is due to hydrodynamic circulation, transport and dispersion. The estimation of the pollutant concentration in the nearshore sea using numerical models, which simulate the forementioned processes, is computationally intensive and requires data, which in many cases are not readily available. A more convenient approach is to use cell models (well-mixed reservoir models).
An application of a cell model is given by Uchiyama et al. (2000 [31]), who investigated the nutrient concentration in the nearshore sea due to SGD. They found that the long-term pollutant concentration depends on the relationship between the pollutant fluxes due to SGD and the exchange flux between the nearshore sea and the open ocean. For the conditions of the investigated case, these authors further found that the volumetric exchange flux between the nearshore sea and the open ocean is three orders of magnitude larger than the SGD flux. Thus, the long term pollutant concentrations, resulting under the assumption that the pollution of the open ocean water is negligible, were found to be of minor importance for the nearshore sea environment.
For estimations of the exchange of water between the nearshore sea (surf zone) and the offshore zone, a relatively simple model presented by Inman et al. (1971 [32]) can be used. The data required are the wave and beach characteristics (breaker height, breaker angle, beach slope in the surf zone, beach slope offshore). For open beaches, typical wave and beach characteristics, it is probable that the water exchange between the surf zone and the offshore strongly dilutes pollutants transported in the surf zone by submarine groundwater discharge, for usual values of SGD (5-50 cm/day) as reported in Burnett et al. (2006 [1]), Gallardo and Marui (2006 [19]) and Gilfedder et al. (2021[33]). However, in semi-closed bays or in nearshore seas with weak wave action and circulation, it can be shown using cell models, that the long term pollutant concentration in the nearshore environment due to the impact of SGD is not negligible. In such cases, measures to reduce the pollutant load of groundwater are required.
See also
- Submarine groundwater discharge in coastal regions: development of a new sampling technique
- Groundwater management in low-lying coastal zones
References
- ↑ 1.0 1.1 1.2 1.3 1.4 1.5 1.6 1.7 1.8 Burnett, W.C., et al. (2006) Quantifying submarine groundwater discharge in the coastal zone via multiple methods. Sciences of the Total Environment, 367 498-543. Cite error: Invalid
<ref>
tag; name "Burnett" defined multiple times with different content Cite error: Invalid<ref>
tag; name "Burnett" defined multiple times with different content Cite error: Invalid<ref>
tag; name "Burnett" defined multiple times with different content Cite error: Invalid<ref>
tag; name "Burnett" defined multiple times with different content Cite error: Invalid<ref>
tag; name "Burnett" defined multiple times with different content - ↑ Michael H.A., Mulligan A.E. and Harvey C.F. (2005) Seasonal oscillations in water exchange between aquifers and the coastal ocean. Nature 436 1145-1148.
- ↑ Sawyer A. H., Shi F., Kirby J. T. and Michael H. A. (2013). Dynamic response of surface water-groundwater exchange to currents, tides, and waves in a shallow estuary. J. Geophys. Res. C 118 1749–1758
- ↑ 4.0 4.1 Kwon, E. Y., Kim, G,. Primeau, F., Moore, W.S., Cho, H.-M., DeVries, T., Sarmiento, J. L., Charette, M. A. and Cho, Y.-K. (2014) Global estimate of submarine groundwater discharge based on an observationally constrained radium isotope model. Geophys. Res. Lett. 41 8438–8444, doi:10.1002/2014GL061574
- ↑ Burnett, W.C., et al. (2003) Groundwater and pore water inputs to the coastal zone. Biogeochemistry 66 3-33.
- ↑ 6.0 6.1 6.2 6.3 6.4 Luijendijk, E., Gleeson, T. and Moosdorf, N. (2020) Fresh groundwater discharge insignificant for the world’s oceans but important for coastal ecosystems. Nature Commun. 11 1260. https://doi.org/10.1038/s41467-020-15064-8
- ↑ Sawyer, A.H., David, C.H. and Famiglietti, J.S. (2016) Continental patterns of submarine groundwater discharge reveal coastal vulnerabilities. Science 353 705–707
- ↑ Smith A.J. (2004) Mixed convection and density-dependent seawater circulation in coastal aquifers. Water Resource Res., 40 W08309. doi:10.1029/2003WR002977
- ↑ Kaleris V. (2006) Submarine groundwater discharge: Effects of hydrogeology and of nearshore surface water bodies. J. Hydrology, 325 96-117.
- ↑ Li L., Barry D. A., Stagnitti F. and Parlange J.-Y. (1999) Submarine groundwater discharge and associated chemical input to a coastal sea. Water Resour. Res. 35(11) 3253-3259
- ↑ 11.0 11.1 11.2 11.3 Taniguchi M., Dulai H., Burnett K.M., Santos I.R., Sugimoto R., Stieglitz T., Kim G., Moosdorf N. and Burnett W.C. (2019) Submarine Groundwater Discharge: Updates on Its Measurement Techniques, Geophysical Drivers, Magnitudes, and Effects. Front. Environ. Sci. 7 141
- ↑ Nakajima, T., Kuragano, M., Yamada, M. and Sugimoto, R. (2024)Comparing nearshore and embayment scale assessments of submarine groundwater discharge: Significance of offshore groundwater discharge as a nutrient pathway. Science of the Total Environment 908 168068
- ↑ Santos, I.R., Chen, X., Lecher, A.L., Sawyer, A.H., Moosdorf, N., Rodellas, V., Tamborski, J., Cho, H-M., Dimova, N., Sugimoto, R., Bonaglia, S., Li, H., Hajati, M-C. and Li, L. 2021. Submarine groundwater discharge impacts on coastal nutrient biogeochemistry. Nat Rev Earth Environ 2: 307–323
- ↑ Slomp, C.P. and Van Cappellen, P. (2004) Nutrient inputs to the coastal ocean through submarine groundwater discharge: controls and potential impact. J. Hydrol. 295 64–86
- ↑ 15.0 15.1 Robinson, C.E., Xin, P., Santos, I.R., Charette, M.A., Li, L. and Barry, D.A. (2018) Groundwater dynamics in subterranean estuaries of coastal unconfined aquifers: controls on submarine groundwater discharge and chemical inputs to the ocean. Adv. Water Resour. 115 315–331
- ↑ Leitao F., Encarnaçao J., Range P., Schmelz R. M., Teodosio M. A. and Chicharo L. (2015). Submarine groundwater discharges create unique benthic communities in a coastal sandy marine environment. Estuar. Coast. Shelf Sci. 163 93–98
- ↑ Welti N., Gale D., Hayes M., Kumar A., Gasparon M., Gibbes B. et al. (2015). Intertidal diatom communities reflect patchiness in groundwater discharge. Estuar. Coast. Shelf Sci. 163 116–124
- ↑ Cho H. M., Kim G. and Shin K. H. (2019). Tracing nitrogen sources fueling coastal green tides off a volcanic island using radon and nitrogen isotopic tracers. Sci. Total Environ. 665 913–919
- ↑ 19.0 19.1 Gallardo A.H. and Marui A. (2006) Submarine groundwater discharge: an outlook of recent advances and current knowledge. Geo-Mar Lett 26' 102-113. Cite error: Invalid
<ref>
tag; name "Gallardo" defined multiple times with different content - ↑ Sekulic, B. and Vertacnik, A. (1996) Balance of Average Annual Fresh Water Inflow into the Adriatic Sea. Water Resources Development, 12(1) 89-97.
- ↑ McDonalt M.G. and Harbaugh A.W. (1988) A modular three-dimensional finite-differences groundwater flow model. US Geol Surv Open-File Report 83-875, 528p.
- ↑ Bear J. et al. (Eds), (1999) Seawater Intrusion in Coastal Aquifers. Concepts, Methods and Practices. Kluwer Academic Publishers, Dordrecht.
- ↑ Smith A.J. and Turner J.V. (2001) Density-dependent surface water-groundwater interaction and nutrient discharge in the Swan-Canning Estuary. Hydrological Proc, 15 2595-2616.
- ↑ Kaleris V. et al. (2002) Modelling submarine groundwater discharge: an example from the western Baltic Sea. J. Hydrology, 265 76-99.
- ↑ Langevin G.D. (2003) Simulation of Submarine Ground Water Discharge to a Marine Estuary: Biscayne Bay, Fl. Ground Water, 41(6) 758-771.
- ↑ Destouni G. and Prieto C. (2003) On the possibility for generic modelling of submarine groundwater discharge. Biogeochemistry 66 171-186.
- ↑ Taniguchi M. et al. (2003) Spatial and temporal distribution of submarine groundwater discharge rates obtained from various types of seepage meters at a site in the northeastern Gulf of Mexico. Biogeochemistry 66 35-53
- ↑ 28.0 28.1 Oberdorfer J.A. (2003) Hydrogeologic modeling of submarine groundwater discharge: comparison to other quantitative methods. Biogeochemistry 66 159-169. Cite error: Invalid
<ref>
tag; name "Oberdorfer" defined multiple times with different content - ↑ Moore W.S. (1996) Large groundwater inputs to coastal waters revealed by 226Ra enrichment. Nature, 380 612-614
- ↑ SCOR-LOIZ (2004) Submarine groundwater discharge management implications, measurements and effects. IHP-VI Series on Groundwater 5. IOC Manuals and Guides 44. United Nations Educational, Scientific and Cultural Organization, Paris
- ↑ Uchiyama Y., Nadaoka K., Roelke P., Adachi K. and Yagi H. (2000) Submarine groundwater discharge into the sea and associated nutrient transport in a sandy beach. Water Resources Res. 36(6) 1467-1479.
- ↑ Inman D.L., Tait R.J. and Nordstrom C.E. (1971) Mixing in the surf zone. J. Geophys. Res., 76 3493-3514.
- ↑ Gilfedder B.S., Waska H., Wismeth F. and Frei S. (2021) Using heat as a tracer to map and quantify water infiltration and exfiltration along a complex high energy beach face. Estuarine, Coastal and Shelf Science 250 107140
Please note that others may also have edited the contents of this article.
|